Viewing the Cosmos
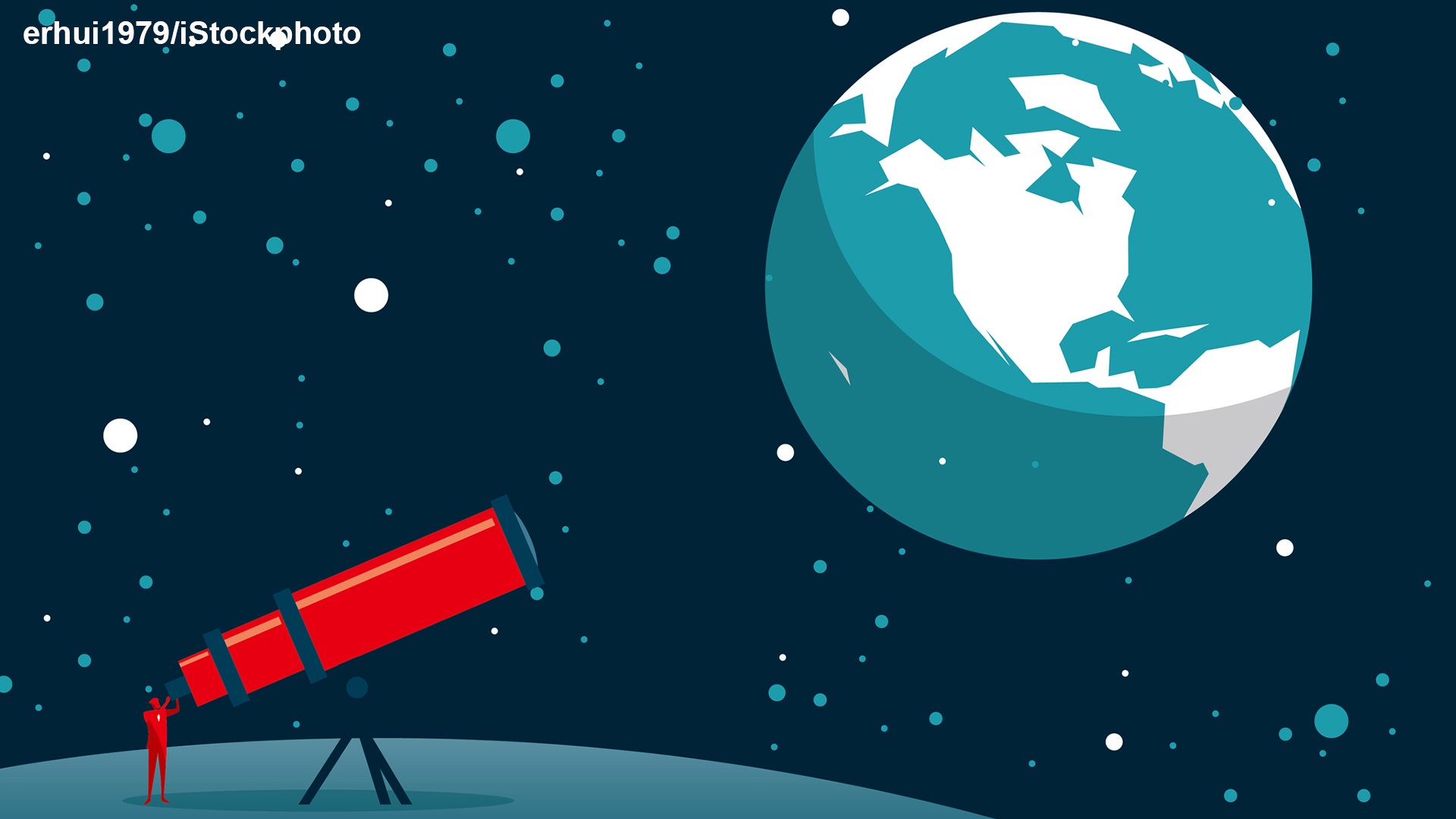
By Ben Skuse
If Galileo Galilei were alive today, he would take comfort in recognising the basic elements that make up the telescopes many of us dust off and set up in our back gardens or windowsills to peer at the moon and planets on a fine, cloud-free night. In contrast, if we were to show him how his modern-day scientific peers view the cosmos, he would quickly come unstuck.
For current astronomers, visible light astronomy is just one small tool in what Galileo would likely deem a cabinet of curiosities. But it is still a very useful tool. The venerable Hubble Space Telescope, which continues to peer deep into the universe over 30 years after its launch in 1990, primarily sees in the visible portion of the electromagnetic spectrum. Circling our planet in geocentric low-Earth orbit, it is a Cassegrian reflector telescope featuring cameras, spectrographs, fine guidance sensors and a 2.4 metre mirror that collect about 40,000 times more light than the human eye. And it has provided rich insights into the cosmos.
In 1998, two separate research teams published results wherein they trained an army of telescopes on several supernovae (explosions at the end of stars’ lives) of a specific type. Type Ia supernovae are a class of supernovae with an expected brightness comparable to the brightness of an entire galaxy, and this brightness is quite standard making it easier to gauge their distance.
The teams expected these supernovae to be brighter than they would have been in a universe expanding at a constant rate, as the popular view in the 1990s was that the universe had been ballooning since the Big Bang but expansion was decelerating. Instead, the supernovae were fainter: expansion was inexplicably accelerating, driven by an unknown form of anti-gravitational energy that was later termed ‘dark energy’. The precision of Hubble measurements of these supernovae was crucial in demonstrating that they were fainter than expected.
For this monumental discovery, the two team leaders Saul Perlmutter and Brian P. Schmidt, and Schmidt’s colleague Adam G. Riess, were awarded the 2011 Nobel Prize in Physics. Today, it is thought around 70% of the energy of the universe is dark energy, but its nature remains a mystery; making it one of the biggest cosmological questions still to be answered.
(00:07:22 - 00:10:33)
(00:06:50 - 00:08:00)
(00:16:57 - 00:18:52)
Another landmark discovery reliant on visible-light measurements was announced in 1995: the first detection of a planet orbiting a star like the Sun. Nobel Prize in Physics 2019 recipients Michel Mayor and Didier Queloz built a spectrograph called ELODIE and used it on the 1.93m telescope at the Haute-Provence Observatory in France to peer at numerous stars in the hope of seeing tiny telltale changes in the colour of a star’s light that indicate a planet is present. They struck gold with 51 Pegasi, a star 50 light years from Earth. The planet – 51 Pegasi b, now officially named Dimidium – is Jupiter-sized and takes four days to complete its orbit at a toasty distance of only 8 million kilometres from its host star.
Not only did 51 Pegasi b’s discovery alter humanity’s perception of its place in the universe, but it also spawned an entirely new field in astronomical research: exoplanet exploration. To date, over 5,000 exoplanets have been confirmed, ranging from Earth-sized rocky planets to gas giants several times larger than Jupiter.
(00:20:06 - 00:23:57)
The most recent Nobel win for visible light astronomy came in the form of a share of the 2020 Nobel Prize in Physics, awarded to Reinhard Genzel and Andrea Ghez for their discovery of Sagittarius A*, a supermassive black hole at the centre of our galaxy.
The heart of the Milky Way is hidden from view due to the dust and cosmic detritus between Earth and the galactic centre. But from the 1990s onwards, two teams led by Genzel and Ghez separately began to peel away this cosmic shroud. They were looking for stars near the centre with peculiar orbits that would indicate they were accelerating as they fell closer and closer to the putative supermassive black hole.
With Genzel’s team using the four 8 metre telescopes of the European Southern Observatory in Chile, and Ghez’s team the Keck telescopes on Mauna Kea in Hawaii, it took until 2008/9 before they could confirm their suspicions. The trajectories of several close-in stars indicated that Sagittarius A* fits 4 million solar masses into a space no larger than the Solar System – a dead giveaway that the Milky Way’s core must contain a supermassive black hole.
(00:17:04 - 00:26:15)
Radio Star
Though these visible-light discoveries prove that the type of astronomy Galileo pioneered is still alive and kicking, stepping beyond the sliver of the electromagnetic spectrum human eyes detect has literally shown the universe in a new light. Arguably the most fruitful slice of the spectrum has been the radio range.
The first extraterrestrial radio signals were picked up accidentally by Karl Jansky in the early 1930s, but radio astronomy really came into its own from the 1950s onwards after breakthroughs in radar technology during World War II. However, it took another two decades for radio astronomy to be recognised by the Nobel Committee, with the first Nobel Prize in Physics dedicated to the discipline awarded in 1974. Surprisingly, it was also the first Nobel for the entire field of astronomy.
Sir Martin Ryle received half the Prize for his development of the aperture synthesis technique, which boils down to using a large number of small radio telescopes spread over a wide distance to achieve the same precision as one vast telescope; a method still employed in many modern radio telescopes today, including the mammoth Square Kilometre Array under construction in Australia and South Africa.
The other half of the Prize went to Antony Hewish for his role in the discovery of pulsars. In 1967, Hewish’s PhD student Jocelyn Bell Burnell detected a radio signal that repeated every one and a third seconds. The researchers jokingly labelled the anomalous signal LGM-1 for “Little Green Men’ initially. It was later interpreted as having come from a rapidly spinning neutron star – where matter is compressed to the extent that electrons and protons recombine to form neutrons – blasting out pulses of radiation at regular intervals. As this confirmed that neutron stars were a real phenomenon, the discovery opened up an exciting new avenue for physicists studying the properties of matter under very extreme conditions.
(00:00:21 - 00:03:14)
(00:00:11 - 00:02:34)
Four years after the first Nobel Prize for research in astronomy, Arno A. Penzias and Robert W. Wilson were awarded for one of the most important discoveries in cosmology in the 20th Century: cosmic microwave background (CMB) radiation. In 1964, the two researchers uncovered a low, steady, persistent noise in their radio telescope data at Bell Laboratories USA, that was evenly spread across the sky. Consulting with cosmologists, the best-fit explanation for this mysterious pervasive static was that it was relic radiation left over from the Big Bang.
The discovery of the CMB was critical evidence for the Big Bang theory; the idea that the universe expanded from an infinitesimally small point to what we see today. At the time, the Big Bang theory was hotly debated and rivalled by other popular ideas, most prominently the steady-state theory which posited that the universe had no beginning or end in time. In effect then, the discovery of the CMB set physicists on the right course for untangling the very origin of the universe.
(00:09:04 - 00:15:57)
Other Signals From the Deep
Other bands of the electromagnetic spectrum have also proved to be rich hunting grounds for exposing new details and knowledge of the universe we live in. For example, alongside cameras that can see in the visible part of the electromagnetic spectrum, Hubble features imagers that read ultraviolet and infrared light. Its successor – the James Webb Space Telescope (JWST) – primarily views the cosmos in infrared. These non-visible bands have allowed Hubble to, for instance, expose an area of new star formation in nearby galaxies and peek at some of the earliest galaxies formed after the Big Bang. And JWST has already returned extraordinary images of exoplanets, dying stars, the formation of galaxies and the birthplace of stars.
(00:20:32 - 00:27:34)
Telescopes dedicated to X-ray imaging have offered unique insights too. Riccardo Giacconi (Nobel Prize in Physics 2002) is regarded as the founding father of X-ray astronomy. In 1962, his team used a small X-ray detector aboard an Aerobee rocket (to get above the confounding effects of the Earth’s atmosphere) to discover Scorpius X-1, the first source of X-rays outside the Solar System. He later worked on the first orbiting X-ray astronomy satellite Uhuru, as well as Einstein Observatory and the Chandra X-ray Observatory launched in 1999. Each of these satellites provided fresh perspectives on the wonders and workings of the cosmos, revealing thousands of distinct X-ray sources, fundamental insights into galaxy formation and evolution, and new ways to investigate the nature of mysterious phenomena like dark matter.
(00:00:02 - 00:12:09)
(00:05:10 - 00:13:54)
(00:05:51 - 00:31:20)
New Messages
As these examples show, Galileo would likely be dumbfounded by the multitude of observational techniques across the electromagnetic spectrum modern astronomers can wield. But if he really thought about it, even though the spectrum of light available has drastically broadened, and telescopes grown tremendously in size and complexity (even being launched into space), the fundamental nature of astronomy – capturing and analysing light from the cosmos using a telescope – did not change for nearly 400 years. That is, until humanity learned to obtain and interpret other signals from space.
Alongside photons (i.e. light), cosmic rays, neutrinos and gravitational waves have gradually been added to the ‘messenger’ signals raining down on Earth that astronomers can detect and analyse to build better understanding of the universe. Each messenger is generated by one of the four forces of nature – photons by the electromagnetic force, gravitational waves by gravity, cosmic rays by the strong nuclear force and neutrinos by the weak nuclear force – meaning their individual presence (or absence) and characteristics provide astronomers with a better grasp of the nature of the objects from which they came.
Particles Packing a Punch
Cosmic rays are not rays at all, but streams of highly energetic protons, nuclei and electrons travelling at near-light speeds throughout the cosmos, some of which rain down on the Earth. First discovered during a series of daring hot-air balloon flights in 1912 by Victor Hess (1936 Nobel Prize in Physics), the most extreme type of cosmic rays are the most energetic particles observed in nature. But what generates them is a mystery, though it is thought that extremely violent astrophysical events such as pulsars, gamma-ray bursts, jets from active galactic nuclei, starburst galaxies, etc, could be potential sources.
The largest cosmic ray detector on Earth – the Pierre Auger Observatory in Argentina – looks nothing like a telescope. It consists of an array of 1,660 surface detectors (large barrels of water) spread across 3,000 square kilometres. The Observatory essentially captures the aftermath molecules of ultra-high energy cosmic rays smashing into the atmosphere and knocking into air molecules to create vast particle showers. Using this technique, in 2017 Pierre Auger researchers reported these cosmic rays appear to originate outside the Milky Way. However, a detection accompanied by other messenger signals is needed to confirm this result.
(00:06:34 - 00:10:54)
(00:12:10 - 00:13:30)
(00:06:00 - 00:08:07)
Ghost Particles
Neutrinos were first hypothesised by Wolfgang Pauli (1945 Nobel Prize in Physics) in 1930 and experimentally detected in 1956 by a team led by Frederick Reins (1995 Nobel Prize in Physics) and Clyde L. Cowan. They are infinitesimally small point-like particles with no electrical charge and almost no mass. About 100 trillion neutrinos stream through our bodies every second, but they are impossible to see and seldom react with matter. Yet this has not stopped neutrinos becoming a significant focus of the astronomy community.
In 1968, Raymond Davis Jr. (2002 Nobel Prize in Physics) led the Homestake experiment in detecting neutrinos from the Sun for the first time. As anyone can also observe the light from the Sun, technically this represents the first ‘multi-messenger’ observation. But the second multi-messenger observation was when multi-messenger astronomy first became useful. In 1987, deep in a Japanese mine the neutrino observatory Kamiokande, led by Masatoshi Koshiba (2002 Nobel Prize in Physics), and two other observatories detected emission from an area in the Large Magellanic Cloud. Two hours later, traditional optical observatories spotted a stellar explosion from the same area. That supernova, SN 1987A, was the first that modern astronomers were able to study with modern techniques and multiple messengers, revolutionising understanding of these cataclysmic events, and eventually providing direct evidence that supernovae create neutron stars.
More recently, the world’s biggest neutrino observatory – the IceCube Neutrino Observatory at the Amundsen-Scott South Pole Station – hit the headlines. IceCube consists of 5,160 detectors buried in a cubic kilometre of ice designed to pick up traces of rare neutrino interactions with matter. In 2017, it detected a high-energy neutrino, dubbed IceCube-170922A. Within four hours, the space-based Neil Gehrels Swift Observatory – capable of detecting gamma-ray, X-ray, ultraviolet and visible wavelengths – identified a blazar called TXS 0506+056 as a possible source, lying 3.7 billion light-years from Earth. In its host galaxy, this supermassive black hole sends out twin jets of high-energy radiation, one of which happens to be aimed at Earth. The Fermi Gamma-ray Space Telescope soon reported that this blazar was flaring, emitting a huge amount of high-energy gamma-rays. Next, the MAGIC ground-based telescope – observing even higher energy gamma-rays – confirmed Fermi’s result. Finally, observations from 20 telescopes detected a blip in electromagnetic emission across the spectrum. Given the high energies and multiple observations involved, National Science Foundation Director France Córdova declared that with this observation: "The era of multi-messenger astrophysics is here."
(00:07:01 - 00:08:30)
(00:00:01 - 00:04:44)
Ripples in Spacetime
The most recent addition to astronomer’s ‘messenger’ toolkit is gravitational waves. Hypothesised by Albert Einstein (1921 Nobel Prize in Physics) as ripples in spacetime in 1916, the first detection of a gravitational wave event was made at the Advanced Laser Interferometer Gravitational-Wave Observatory (LIGO) in the US. This vast experiment consists of two large L-shaped observatories – one in Pasadena, California, and the other in Cambridge, Massachusetts – that use mirrors spaced four kilometres apart to detect tiny stretches and compressions of spacetime of the order of one thousandth the size of a proton. In 2015, it registered a hit. Named GW150914, the event was triggered by two black holes merging 1.3 billion years ago.
Despite the detection being a triumph for science, the news was not transformational for all astronomers. Black holes were still black and therefore invisible to telescopes. This all changed the same year when the key contributors to the first gravitational wave detection – Rainer Weiss, Barry C. Barish and Kip S. Thorne – were recognised with the 2017 Nobel Prize in Physics. Scientists working at LIGO (and sister observatory Virgo in Italy) detected a neutron star merger for the very first time, named GW170817. Immediately alerting the astronomy community, space-based observatories Fermi and Swift were focused on the area from which the gravitational waves originated, registering a short gamma-ray burst. And further ground- and space-based visible-light observations recorded a short, bright burst of electromagnetic radiation, dubbed a kilonova. In a press release accompanying the announcement, Laura Cadonati, deputy spokesperson for the LIGO Scientific Collaboration, said: “This detection has genuinely opened the doors to a new way of doing astrophysics. I expect it will be remembered as one of the most studied astrophysical events in history.”
(00:10:41 - 00:12:43)
(00:01:00 - 00:04:23)
(00:06:20 - 00:07:12)
(00:11:46 - 00:14:55)
(00:15:55 - 00:19:20)
As these events have shown, by using several messengers in conjunction, astronomers and physicists can wield a formidable toolkit for probing the highest energies and densest, most violent corners of the cosmos. Though multi-messenger events are presently still rare, the precision and power of observatories searching for each of the four messengers are growing, undoubtedly making it more likely that such events will soon become commonplace. And when they do, physicists will have the ideal cosmic laboratory at their disposal to put their physical theories of the universe to the most extreme and exacting tests imaginable. The huge international collaborative nature of this work, and the advanced technologies and concepts would boggle the mind of Galileo, but the underlying motivation – to understand the universe in which we all reside, by looking up – will continue to burn just as brightly in this new era as it did for the father of observational astronomy.