Prof. Dr. Sir Harold Walter Kroto > Research Profile
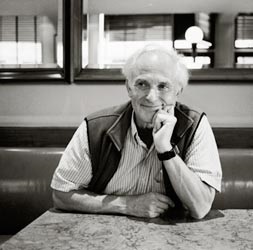
by Luisa Bonolis
Sir Harold Kroto
received the Nobel Prize in Chemistry 1996 together with Robert F. Curl Jr. and Richard E. Smalley for "their discovery of fullerenes".
Becoming a spectroscopist
Harold Kroto was born in Wisbech, a very small town in Cambridgeshire, in October 1939. His parents had moved to Britain as refugees from Germany in the late 1930s, because his father was Jewish. He originated from Poland and in 1955 decided to change his surname Krotoschiner to the shorter form Kroto. At that time, he set up a small factory making balloons and printing them. As a boy, Kroto spent much of his school holidays working at the factory to fill in everywhere, an activity that he later considered an outstanding training ground “for the development of the problem solving skills needed by a research scientist.” He was sent to Bolton School, a school with exceptional facilities and teachers, where he particularly enjoyed art, geography, gymnastics and woodwork. At that time, Kroto liked to play endlessly with a Meccano set, which he credits - among other things - with developing skills useful in scientific research.
At secondary school, he developed an interest in chemistry, physics and mathematics and these became his specialist subjects in the 6th form. He became so fascinated by chemistry - particularly organic chemistry - that he was encouraged by his sixth form teacher, Harry Heaney, to enrol to Sheffield University, the British educational institution that reputably had the best chemistry department in the United Kingdom. As the university course progressed, Kroto started to get interested in quantum mechanics and became especially intrigued by spectroscopy, so that after graduating with first class honours in Chemistry in 1961, he decided to do a PhD in the high-resolution spectroscopy of free radicals produced by flash photolysis, the chemical decomposition by the action of radiant energy.
In 1964, Kroto moved abroad for a while and got a postdoctoral position at the National Research Council in Ottawa, where Gerhard Herzberg had created a Mecca for spectroscopy with a group of excellent collaborators. In 1965, after working for a year in Don Ramsay's laboratory, Kroto developed a new fascination for microwave spectroscopy. He then transferred to Cec Costain's laboratory, where he really learned quantum mechanics following an intensive course. As he recalled in his autobiography, he gradually realised that, “many in the field were stronger at physics than chemistry and in retrospect I subconsciously recognised that there might be a niche for me in spectroscopy research if I could exploit my relatively strong chemistry background.”
Carbon chains in interstellar medium
In 1966, Kroto was offered a postdoctoral position at the University of Sussex in the United Kingdom, but he was interested to move to the US and managed to get a position at Bell Labs to carry out research in quantum chemistry, specifically on liquid phase interactions by laser Raman spectroscopy. During the year, he learned that the postdoctoral position at the University of Sussex in Brighton was still available and he decided to go back to the UK where he was soon offered a permanent lectureship.
By 1970, he had carried out research in the electronic spectroscopy of gas phase free radicals and rotational microwave spectroscopy, had built He-Ne and argon ion lasers to study intermolecular interactions in liquids, carried out theoretical calculations and learned to write programs. He built a microwave spectrometer to start to do photoelectron spectroscopy and at the same time applied for a Hewlett Packard microwave spectrometer, a most advanced device. However, the equipment was placed at Reading, where he had to travel each month with his group. Only in 1974 did he succeed in having his own instrument at Sussex.
The first molecule they studied on the newly arrived Hewlett Packard spectrometer was the carbon chain species HC5N, a member of the group of chemicals with the chemical formula HCnN (with n = 3, 5, 7...) called cyanopolyynes. The start of his interest in molecules containing chains of carbon atoms with numerous multiple bonds can be traced directly to this molecule. Kroto had been fascinated by the chemistry of carbon since his time as a graduate student at Sheffield. Carbon is one of the elements most abundant in nature. It is essential for living organisms and, as an element, occurs in several forms and structures. Various forms of carbon, including graphite, diamond and hydrocarbon molecules, had been intensively studied since the beginning of the 20th century. In 1924, John Bernal had successfully identified the crystal structure of graphite, the most stable form of carbon under standard conditions, owing its name to the ability to leave marks on paper and other objects. Diamonds have been successfully grown synthetically since 1955 and diamond thin films by chemical vapour deposition have become a twenty-first-century material.
In 1975, microwave measurements with his colleague David Walton on long linear carbon chain molecules of the type HCnN, coincided with advances in the detection of molecules in space by radio astronomy. One of the most exciting advances in astrophysics in those years was provided by the ability to detect molecules in space by radio and infrared techniques. After the discovery of ammonia in Orion by Townes and co-workers, microwavers and radioastronomers joined forces and showed that the vast dark clouds that lie between the stars harbour many different types of molecules. Cyanopolyynes were first discovered in interstellar molecular clouds in 1971. They were all identified by the detection of their microwave rotational emission spectra using millimetre wave and microwave telescopes. HC3N was also detected and when Kroto and his group made HC5N he began to wonder whether it might also be present in interstellar space.
These ideas led to a search for HC5N in space with Kroto's friend Takeshi Oka and his collaborators. In 1978, its successful detection in the giant molecular cloud SgrB2 towards the galactic centre came as a surprise, because the molecule turned out to be much more abundant than expected, instigating further work with Colin Kirby on HC7N. The recording of its microwave spectrum enabled a radio search for this species, leading to its surprising radio detection in the Taurus constellation. As recalled by Kroto, “In a moment of considerable drama, the data obtained at Sussex was transmitted to Canada by telephone and found to match exactly the oscilloscope trace associated with the vital signal.” This result attracted wide attention because, at the time, HC7N was the most complex molecule unambiguously to be identified in space. Subsequently HC9N and HC11N were detected in the same way by other investigators, even if funding and other difficulties precluded preparation of these higher cyanopolyynes by conventional laboratory methods at Sussex. The observation of these molecules in the interstellar medium provided evidence for the existence of efficient synthetic mechanisms in operation in interstellar clouds. Many molecules appeared to form in the cold interstellar medium by ion-molecule reactions. However, the ion-molecule reaction theories of the time, which accounted almost perfectly for almost all the other species observed, were just not able to account for the high abundances of such long carbon chains. It seemed to Kroto that some alternative source was needed and fortunately a possible answer started to appear. Cool, carbon-rich, red giant stars, which are constantly puffing out copious quantities of dust, seemed more plausible sources, as Kroto proposed in 1981.
In 1984, Kroto met Robert Curl, a long-time friend, at a conference on molecular structure in Austin, Texas. Curl invited him to visit his laboratory at Rice University in Houston, and introduced him to Richard Smalley, who had pioneered supersonic beam laser spectroscopy, which soon became one of the most powerful techniques in chemical physics. Smalley explained to him how a pulsed laser, focussed on a metal disc, vaporised material, producing atoms which were swept up by pulses of helium, causing clustering and concomitant cooling. Clusters of any element could be studied with Smalley's device, whose intense laser pulse was generating temperatures reaching tens of thousands of degrees, hotter than the surfaces of most stars and certainly hot enough to vaporize any known material. By expansion through a nozzle into a vacuum chamber, the clusters were cooled and the resulting beam was analysed by a time-of-flight mass spectrometer, allowing for a determination of the cluster mass distributions. Smalley and Curl had been making atomic clusters from semiconducting elements like silicon, but Kroto immediately wondered whether, by substituting the metal target disc by a graphite one, the plasma produced by the laser might simulate the dense, circumstellar shell of cool red giant carbon stars and produce the carbon chains. Kroto thus became convinced that Smalley's apparatus was the key to explore the possibility that longer cyanopolyyne chains might be forming in the carbon-rich winds blowing from giant stars. Proving a circumstellar source seemed a wildly exciting prospect. This would give strong support for the stellar solution to the chain problem and provide an important alternative mechanism to the ion-molecule scheme.
To Kroto, it sounded like a dream come true, and he was very keen to start collaboration; Smalley, however, was not intrigued at the beginning. Curl favoured such collaboration, but at the moment it didn't fit in with their research priorities because he and Smalley were busy studying semiconductor clusters. Kroto was soon disappointed in learning that a team of scientists at the Exxon Corporate Research Science Laboratory in Annandale, New Jersey, had just performed experiments using lasers to vaporise graphite to form carbon clusters. Eric Rohlfing, Donald Cox, and Andrew Kaldor had used a machine built for them by Smalley, finding a new family of carbon clusters with more than 30 atoms but, surprisingly, with only even numbers of atoms. However, they did not follow up on this finding.
Only a year later, in August 1985, Smalley finally agreed to give Kroto's idea time on his machine. Kroto was again very excited when Curl called him to say that his experiments were to be carried out at Rice imminently and asked whether he was interested in coming to Houston.
The discovery of fullerenes, a third allotrope of carbon
On September 1, 1985, Kroto's project to simulate carbon-star chemistry was initiated at Smalley and Curl's laboratory, with graduate students Jim Heath, Sean O'Brien and Yuan Liu. To re-create conditions near red giant stars, they vaporised carbon in an atmosphere heavy with hydrogen and nitrogen. Almost immediately, they detected the linear molecules with 5 to 9 carbon atoms that had been observed in space. This showed that Cn (n<30) clusters did indeed react with H and N to form polyynes, such as HC7N and HC9, a result satisfyingly consistent with the idea of a stellar source of interstellar chains. But along with Kroto's long carbon chains, they also observed something completely unexpected. On September 4, using helium as the carrier gas instead of hydrogen and nitrogen, they immediately noticed that something quite remarkable was taking place, an odd peak in the mass spectroscopy measurement of the molecules that formed in the vapour. The peak occurred at a molecular weight of 720, corresponding to an aggregate of exactly 60 carbon atoms and was accompanied by a smaller peak popping up at a higher mass, corresponding to 70 carbon atoms. Yuan Liu noted in the lab book, “C60 and C70 are very strong,” adding an exclamation mark and underlining the entry. Since Kroto was scheduled to return to England early the following week, Heath and O'Brien, the graduate students, worked over the whole weekend to probe the mystery molecule's properties.
One thing became apparent. C60 had unique properties: it formed very readily and exhibited extraordinary stability. No matter how the researchers varied the conditions of the experiment, the C60 peak was invariably there, and in one case the machine had produced 40 times as much C60 as any other carbon cluster. That the peak was so prominent also meant that the molecule was chemically inert. It did not react with other molecules, which suggested it had no dangling bonds. All known carbon-containing molecules have edges that terminate with other elements; even benzene, a very stable ring of carbon atoms, has some edges, which are all terminated with hydrogen bonds. A flat 60-atom hexagonal graphene sheet should add on ca. 20 or more H atoms. But it was clear that C60 did not need hydrogen, or any other element, to tie up its bonds.
Gradually a consensus developed that what might have happened was that flat hexagonal sheets had either formed or ablated from the surface of the graphite disc and closed into a cage, eliminating the reactive edge, but nobody knew how. A closed structure, a cage, would have no dangling bonds, with the valences of every atom satisfied. By symmetry, every atom is equivalent so that there is no specific point of chemical attack. The following Monday, during a last meeting, Buckminster Fuller and his famous geodesic spherical – or nearly spherical - shell structures had been mentioned. Kroto remembered a visit to Expo67 in Montreal where Buckminster Fuller's dome had dominated the horizon. He was a graphic artist in his spare time and as a student he had contemplated becoming an architect and studying with Buckminster Fuller. He had always been fascinated by Buckminster Fuller’s work and he seemed to remember how a toy cardboard “stardome” skymap that he had once built for his children had pentagonal facets as well as hexagonal ones. And actually, the Montreal dome designed by Fuller had a precursor in the Zeiss Planetarium realised in Jena in 1923, hosting the first sophisticated mechanical-optical device for producing a realistic image of the starry sky. They looked at a photograph of one of Fuller's domes, but the structure's grid appeared to be entirely composed of hexagons. Kroto was going to fly back to the UK the next day, and that evening he invited the team out for dinner to a Mexican Restaurant to celebrate the exciting discovery of C60. During the meal they spent the whole time trying to solve the puzzle. Smalley wondered how a sheet of hexagons could close and decided that the only way to find out was to build one. He worked at his computer during that same night trying to generate a structure, but he failed and so he turned to paper, tape and scissors. He began by cutting out hexagons, but soon found that he was not able to close the structure in any way. During a pause, drinking a beer, he remembered the stardome Kroto had mentioned, which he made using both pentagons and hexagons. He went back to his desk and was astonished to see that by interspersing pentagons among the usual carbon hexagons (many carbon compounds have actually both five- and six-membered rings) the result would be a geodesic dome with sixty vertices. Quickly doubling the hemisphere, Smalley saw an exquisitely symmetrical sphere. He had stumbled upon a mathematical truth, which Fuller employed in his domes: a sheet of hexagons can be made to curl by adding pentagons. Sixty, it turned out, was the only number of atoms that could form a nearly perfect sphere.
Cages of graphene sheets had already been proposed by David Jones in 1960 and had later been discussed in more detail in a book in 1982, where he remarked that pentagons would serve in a hexagonal lattice to produce a complex curvature. Jones was influenced by D. W. Thompson, who had pointed out, in his most famous book On Growth and Form of 1942, that though a sheet of hexagons may warp and bend, it can never close. Kroto learnt from Jones's book about the necessity for 12 pentagons in any closed-shell hexagonal structure. The C60 structure had been in fact proposed without guidance from Euler's rule: Euler's network closure requirement states that for any convex 3-dimensional polyhedron the number of faces plus the number of vertices minus the number of edges always equals 2. This general rule was noticed by Descartes in 1620 and later proved by Euler in 1752 and since then has been known as Euler's theorem. Euler's formula can be used to determine whether it is possible to form a closed shape out of a given set of faces. It was realised by the ancient Greeks that there are only three polygons that can form closed shapes on their own - that is, a square a triangle and a pentagon. According to Euler's theorem 12 pentagons are required for closure of the carbon network consisting of n hexagons. It can be proved that there are exactly 12 pentagons in the closed cage, irrespective of the number of hexagons and using more hexagons simply makes the cage larger. C60 is the first stable “cage-like molecule” of this kind because it is the smallest one to obey this rule, having 20 hexagons. Pentagonal rings are defects as far as carbon bonding is concerned, so that having adjacent pentagons would introduce a great deal of strain and decrease the stability of the molecules. The extraordinary combination of chemistry and structural symmetry dictates the final structure of these molecules, which must have an even number of carbon atoms. Remarkably, C60 had been again predicted, first in 1970 by Eiji Osawa - who was considering the structure of corannulene, a polycyclic aromatic hydrocarbon with the chemical formula C20H10, a molecule consisting of a central pentagon of carbon atoms surrounded by five hexagons. Osawa glanced at his son's soccer ball and recognised the same pattern. Actually this molecule can be considered a fragment of a cage molecule. Osawa and Yoshida reported the idea in a Japanese scientific journal, but it did not reach Europe or the United States. In 1970, R. W. Hensen proposed the structure and made a model of C60, but the results were never published. It is noteworthy that about the same time, in 1973, two Russian chemists, Bochvar and Gal'pern, made a quantum-chemical analysis on the stability of C60 performing calculations and giving the correct picture of its electronic structure. They named it carbo-s-icosahedrene and published their proposal in the Proceedings of the USSR Academy of Sciences. A stable icosahedral molecule C60 had been also theoretically predicted in 1983 by A. D. J. Haymet, who had called it “footballene”.
The next day Smalley tossed the paper model on a table in his office. They were all ecstatic and overtaken to see the beauty of C60. It was an icosahedron with 60 vertices, 12 pentagonal and 20 hexagonal faces. The icosahedron, a polyhedron with 20 faces, is one of the five regular Platonic solids. It was drawn by Leonardo da Vinci and included in the treatise De Divina proportione by Luca Pacioli. After all, recalled Kroto in his Nobel Lecture, “the molecule was so beautiful that it just had to be right.” Within minutes they were able to make sure that the bonds on the structure obeyed the rules of carbon bonding. Smalley called Bill Veech, chair of Rice’s mathematics department, to ask if he was familiar with the form. The answer came a few minutes later in a return call: “I could explain this to you in a number of ways, but what you’ve got there, boys, is a soccer ball.”
This was not the first time that Buckminster Fuller's geodesic domes had helped researchers to understand the structure of matter. In the early 1960s they had inspired Donald Caspar and Aaron Klug to develop the principle of quasi-equivalence in virus research. With sufficiently high resolution X-ray and electron microscopy they found in more than one case that what appeared to be spherical viruses, actually displayed full icosahedral symmetry, arguably the highest symmetry shown in Nature. In 1956, Crick and Watson had proposed that small isometric viruses should form an isometric shell around the nucleic acid. The largest number of subunits that could be arranged symmetrically on the surface would be 60, with icosahedral symmetry, as was indeed indicated by X-ray patterns and electron microscope images. However, Caspar and Klug found that the number of subunits in most virus shells was considerably larger than 60. How to resolve the dilemma of the virus shells? In reading about Buckminster Fuller's structures their attention focused on his tensegrity spheres, in which the engineering principle of continuous tension and discontinuous compression allowed domes to deploy a lightweight lattice of interlocking icosahedrons that could be skinned with a protective cover. Following this architectural design, they imagined the virus shells spread out as flat hexagonal networks that could be cut and folded to form larger and larger icosahedra and proposed that the equivalent bonding required by exact symmetry could be relaxed to what they termed 'quasi-equivalence' and thus accommodate some multiples of 60 subunits. The concept of self-assembly underlying the principle of quasi-equivalence that had helped to interpret the structures of spherical viruses materialised in the surface patterns recognised in photographs taken through an electron microscope. For his development of crystallographic electron microscopy and his structural elucidation of biologically important nucleic acid-protein complexes, Klug was awarded the Nobel Prize in Chemistry 1982.
Kroto suggested calling the molecule Buckminsterfullerene and after some discussion the name was accepted. He rescheduled his flight back to the UK for the next Wednesday in order to write up the paper announcing the discovery. The experiments had started on September 1, and on September 13, Kroto, Curl, Smalley, Heath and O'Brien sent a two-page letter to Nature with the title “C60: Buckminsterfullerene.” It was published in the 14 November issue of the journal showing a photograph of a football (in the United States, a soccer ball) on Texas grass and explaining how the C60 molecule featured in the article was suggested to have the icosahedral structure formed by replacing each vertex on the seams of such a ball by a carbon atom.
The observation that C60 is formed spontaneously in condensing carbon vapour was greeted with some doubt by several members in the chemistry community. It seemed incredible that this highly symmetrical, closed, low entropy molecule was forming spontaneously out of the chaos of condensing high-temperature carbon vapour. Seeing a line in a mass spectrum did not convince all scientists of the discovery according to which there were now three known forms of pure carbon: the network solids, diamond and graphite, and a new class of discrete molecules - the fullerenes. These cage-like molecules constituted the third carbon allotrope, with C60 the archetype and the roundest molecule that can possibly exist.
As Smalley duly remarked, “That they eluded discovery until so recently is a reminder that some of nature's most subtle but fundamental phenomena can still be found right under our noses.” As it was soon discovered, fullerenes exist everywhere in nature. They exist near carbon stars, in interstellar space, in volcano craters and especially in places where carbon and high energy exist. This totally new molecular form was hiding even in gas burning in a household gas cooker or in the flame of a regular lighter, but it kept hidden for a long time, waiting for the scientific collaboration between spectroscopists and chemists to be revealed for the first time to the world, as the final step of decades of research and developments of methods to study matter at different scales.
The fullerenes offered a challenge to theoretical chemistry. Although carbon had always been subjected to far more study than all other elements put together, the buckminsterfullerene hollow-cage structure, proposed to account for the exceptional stability of the C60 cluster, was now shedding a totally new and revealing light on several important aspects of carbon's chemical and physical properties that were quite unexspected and others that were not previously well understood. During the period 1985-1990, the Curl/Smalley team at Rice and Kroto at Sussex managed to amass a wide range of circumstantial evidence to support the fullerene structure proposal. A first experiment had successfully tried to trap metal ions inside the molecule in order to prove that it had a cage structure. In addition to C60, another molecule, C70, appeared to be quite special in their early experiments. C70 has 25 hexagons, producing a shape reminiscent of a rugby ball. In fact, they found that all the even-numbered carbon clusters larger than about 32 atoms were remarkably stable (although less so than 60 or 70), and the evidence soon led them to postulate that all these molecules had the structure of geodesic domes.
However, although their evidence was sound and their conclusions were supported by extensive further experiments and theoretical calculations, they could not collect more than a few tens of thousands of these special new molecules. This amount was plenty to detect and probe with the sophisticated techniques available in their laboratory, but their evidence was indirect and there was not enough to see, touch, or smell. Thus for five years they searched for a method of producing visible amounts of fullerenes. In the end, the breakthrough was made not by chemists but by physicists working in a totally different area. Wolfgang Krätschmer, of the Max Planck Institute for Nuclear Physics in Heidelberg, Germany, and Donald Huffman of the University of Arizona, with their collaborators, had been engaged for decades in a study of interstellar dust, which they assumed to consist mainly of particles of carbon (the most common particle-forming element). They had developed laboratory experiments, which focused on the composition of the particulate carbon material that occupies the interstellar medium. They modelled the interstellar dust in the laboratory by vaporising carbon and condensing it in as many ways as possible. Full acceptance of buckminsterfullerene came in 1990, when Krätschmer and Huffman, with their students Konstantinos Fostiropoulos and Lowell Lamb, eventually succeeded in synthesising C60 in sufficient quantities to allow structural characterisation using their electric carbon-arc discharge apparatus.
This breakthrough triggered an explosion of research. Fullerenes could now be produced in sufficient quantity to permit unequivocal verification of their chemical existence and their remarkable chemical and physical properties. Smalley then turned toward the “big brother” of the buckyball, the carbon nanotube, which was reported initially by Sumio Iijima in Japan and others in 1991, and which Smalley had predicted from chemical geometrical arguments in the late 1980s. Tubular carbon filaments had been described already in 1952 by two Russian researchers, L. V. Radushkevich and V. M. Lukyanovich, whose paper was published in Russian during the cold war and received little attention in the West. Carbon nanotubes, the cylindrical cousins of buckyballs that can be considered as elongated fullerenes, have since then revealed unique and unusual properties that led to the development of completely new kinds of advanced materials.
In 1991, Hiijima reported the existence of nested carbon nanocages (now known as graphitic onions), hollow graphitic cages similar to fullerene structures that can be multilayered and have irregular shapes, and eight years later Kroto and Ken McKay also proposed the model of graphitic onions consisting of nested icosahedral fullerenes containing only pentagonal and hexagonal carbon rings (C60, C240, C540, C960...). All this marked the beginning of the science of fullerenes, a new era for the carbon allotropes, full of most surprising results.
In 1996, Curl, Kroto, and Smalley were awarded the Nobel Prize for Chemistry "for their discovery of fullerenes." Since then research on fullerenes has resulted in the synthesis of a steadily increasing number of new compounds, already more than one thousand. Nowadays, C60 molecules are used as the basis of a new type of chemistry - fullerene chemistry - in which various types of organic, inorganic and organometallic molecules have been reacted with these carbon cages. When C60 crystals are doped with alkali metals, such as potassium, caesium or rubidium, it is possible to obtain superconductors below 33 K. Among the strange properties of C60, even inhibition of the HIV virus has been detected. Unlike bacteria, which are much larger, viruses are actually small enough to be inhabitants of the nanoworld. Twenty-five years after their discovery, fullerenes provide abundant research opportunities in pure chemistry, materials science, pharmaceutical chemistry, and nanotechnology, having become an important part in the worldwide development of smart materials in the new millennium.
In 2010, molecules of C60 and C70 were found in space for the first time using NASA's orbiting Spitzer infrared telescope. As soon as they were discovered, it was in fact suggested that their extreme stability, in particular against photodissociation, makes fullerenes ideally suited to survive the harsh radiation field in the interstellar medium and for this reason scientists persisted in looking for them in space for decades. The molecules were accidentally found - and in great numbers - by astronomers studying the young planetary nebula Tc1. The data from the spectrometer matched laboratory spectral fingerprints of the two molecules perfectly. They were the largest molecules ever found in space. Following this discovery, C60 has been detected in many different environments showing that, once injected into the interstellar medium, molecules of this stable species survive and are thus likely to be widespread in the Galaxy, where they contribute to many different processes and complex chemical reactions that are currently being studied by several investigators.
Bibliography
American Chemical Society National Historic Chemical Landmarks. Discovery of Fullerenes. http://www.acs.org/content/acs/en/education/whatischemistry/la
Curl F. and Smalley R. E. (October 1991) Fullerenes. Scientific American: 54-63
Kroto H. and D. R. M. Walton. (1993) Polyynes and the Formation of Fullerenes. Philosophical Transactions: Physical Sciences and Engineering 343 (1667): 103-112
Kroto H. (1996) Autobiography. Nobel Prize. http://www.nobelprize.org/nobel_prizes/chemistry/laureates/1996/kroto-bio.html
Kroto H. (1998) Space, Stars, C60, and Soot, Science 242 (4882): 1139-1145
Smalley R. E. (1991) Great Balls of Carbon: The Story of Buckminsterfullerene. The Sciences 31 (2): 22-28
Taubes G. (1991) The Disputed Birth of Buckyballs. Science 253 (5027): 1476-1479
Wade A. (2008) Smalley, Richard Errett. Gale Virtual Reference Library. Web. 17 Oct. 2014. http://go.galegroup.com/ps/i.do?id=GALE%7CCX2830906099&v=2.1&u=mpi_vb&it=r&p=GVRL&sw=w&asid=0a0f30ab42dafbf9eac5314e5207d2a5