The Brain and How It Works
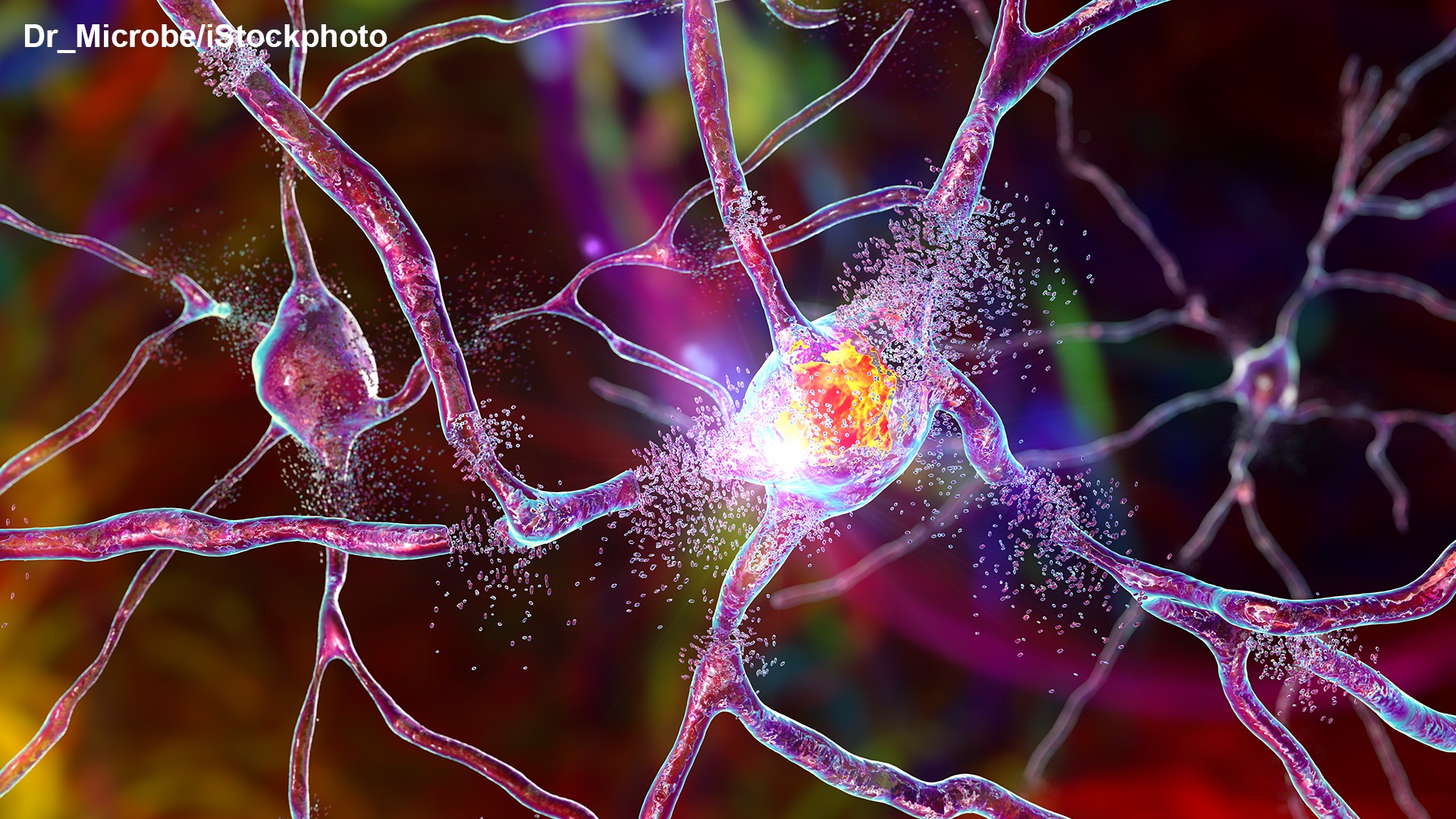
by Neysan Donnelly
Brain Function 101
The age of modern neuroscience has been ushered in with the brilliant work of the Spaniard Santiago Ramón y Cajal. His amazingly intricate and beautiful depictions of the nervous system laid the basis for the identification and characterisation of many important brain structures and, together with the Italian Camillo Golgi, he was awarded the Nobel Prize in Physiology or Medicine in 1906. Listening to contemporary Nobel Laureates talking at the Lindau Meetings, it is striking how often Cajal’s name comes up and how many still acknowledge their debt to him.
In this spirit, laureates visiting Lindau often provide their audiences with basic insights into the function of the brain and of its functional units, neurons, before discussing the intricacies of their Nobel Prize-winning research. What sets neurons apart from other cells and how do they communicate with each other? Here is a succinct summary by Nobel Laureate Erwin Neher:
(00:02:49 - 00:04:41)
The complete video is available here.
Communication between neurons – the basis of brain function – is achieved through so-called action potentials or nerve impulses that arise through rapid changes in the cell membranes. These changes are enabled by ion channels, proteins that form pores through cell membranes allowing electrically charged molecules or ions to pass through. Thus, ion channels can be said to participate in all brain tasks, from thinking to breathing to moving.[1] For their characterisation, Erwin Neher shared the 1991 Nobel Prize in Physiology or Medicine. In this excerpt from his talk in 2018, Neher talks about what first drew him to these crucial proteins:
(00:03:15 - 00:04:52)
The complete video is available here.
Characterising the function of ion channels would not have been possible without the so-called patch clamp technique, which Neher and Sakmann developed to allow the study of ionic currents in individual cells. In 2018, Neher also briefly discusses the principles and development of the method:
(00:12:04 - 00:14:50)
The complete video is available here.
Based on how they are opened, ion channels come in two ‘flavours’: voltage-gated ion channels open or close based on changes to the voltage gradient that are caused by fluctuations in ion concentrations close to the membrane, while so-called ligand-gated ion channels are regulated by molecules such as neurotransmitters that bind to the channels and induce them to open to allow ions through.[1] For his work on the release and uptake of the catecholamine class of neurotransmitters, which includes adrenaline and dopamine, Julius Axelrod was awarded a share of the Nobel Prize in Physiology or Medicine in 1970. In his talk from a visit to the Lindau Nobel Laureate Meetings in 1970, Axelrod gives a general introduction into how neurotransmitters work and how they elicit action potentials.
(00:01:14 - 00:02:59)
The complete video is available here.
Moving Through Space
The transmission of signals from one neuron to another is facilitated by the synapse. Interestingly, much of what we know regarding how these structures function was actually discovered in the context of neuronal communication with muscles rather than within the brain. Indeed, one of the most important tasks that our brain carries out is telling our muscles to move. The experiments of the Italians Luigi Galvani and Alessandro Volta performed over 200 years ago showed that muscle movement is based on electrical signals.[2] How does this work at a molecular level and how does the brain direct muscles to move? Bernhard Katz shared the Nobel Prize in Physiology or Medicine with Julius Axelrod and Ulf Keuler in 1970 for working out precisely these questions. In his talk at the 2014 meeting, Erwin Neher briefly describes what synapses are and how neuronal communication with muscles leads to muscle contraction.
(00:05:30 - 00:07:43)
The complete video is available here.
Being able to move is obviously crucial, but such a skill would not be much use without the ability to map space, choose direction and measure the speed of movement. These are skills that we take so much for granted that we generally don’t give them any serious thought. However, these fundamental everyday activities rely on hugely sophisticated operations involving different parts of the brain that work together to act like a navigation system.[3] For the discovery and characterisation of this system, John O’Keefe, May-Britt Moser and Edvard Moser were awarded the Nobel Prize in Physiology or Medicine in 2014. The Mosers talk listeners through the discovery of this navigation system and give other fascinating insights into their science in their Nobel Lab 360°.
How does the brain represent space and how was this discovered? John O’Keefe started to focus on the hippocampus, a brain region that was highly interesting to him because it was thought to be involved in memory. However, based on what are now landmark experiments that O’Keefe performed with his student Jonathan Dostrovsky, he concluded that the hippocampus was actually doing something else.[3] Edvard Moser, who shared the 2014 prize with O’Keefe and with whom he has closely collaborated, picks up the story:
(00:05:31 - 00:08:57)
The complete video is available here.
These experiments subsequently led O’Keefe and his collaborator Lynn Nadel to propose that the hippocampus constitutes a cognitive map in which the aforementioned place cells represent separate locations in the environment.[3] Knowing where you are and where you should go involves a lot of brain power, however, not only the hippocampus. Where do the place cells receive their signals from? This was what motivated the Mosers when starting their lab. They discovered that the entorhinal cortex, an area of the brain now known to function as a hub in many important cerebral processes such as memory and time perception, is responsible. Thus, they measured brain activity from that region.
(00:10:55 - 00:12:30)
The complete video is available here.
Grid cells coexist with head-direction cells or “compass cells” discovered by Jim Ranck in the entorhinal system as well as border cells that fire specifically along local borders (intermingled along the grid cells), and speed cells whose firing rates increase proportionally with running speed. Many of these insights were made in experiments where rats were navigating in empty spaces. However, in the real world, we of course have to make our way through spaces that are full of all kinds of different objects. How do we navigate such terrains? Well, it turns out we also have cells for that. The Mosers have very recently discovered a new type of cell in the entorhinal cortex that are responsible for defining our relationship to significant objects in our environment.[4]
(00:21:24 - 00:23:44)
The complete video is available here.
How the Brain Measures Time
Time is also encoded in another region of the entorhinal cortex, the lateral entorhinal cortex. In contrast to the representation of space, which is essentially ‘all or nothing’ firing of grid cells and other position-determining cells, time is expressed in the activity of many individual cells from this region. Describing the recent experiments of a postdoctoral researcher in his lab, Moser shows how the activity or firing of cells in this region changes over time at multiple scales. That is, some cells in the lateral entorhinal cortex ramp up their activities each time over the course of a discrete episode, in this case while rats explore a maze, while, for example, the activity of other cells decreases steadily over the course of multiple episodes.[5]
(00:25:42 - 00:27:45)
The complete video is available here.
As mentioned at another point in the lecture, as well as in the Mosers’ Nobel Lab 360°, this type of measurement is not the absolute circadian representation of the passing of time but rather the time relative to events.
Visual Processing
We talk about our eyes as being our instruments of vision, but considering the amount of brain computation involved, it might be more appropriate to say that it is our brain that ‘sees’. The Swede Torsten Wiesel shared the 1981 Nobel Prize in Physiology or Medicine with David Hubel for their work into visual processing in the brain, i.e., how specialised cells in the visual cortex convert the outside world into the images that we perceive. In this excerpt from his lecture in 1990, he describes the reductionist approach that they took.
(00:03:57 - 00:04:47)
The complete video is available here.
Wiesel and Hubel elucidated several key aspects of how image processing happens in the brain, including the revelation that the angle of incoming light determined if cells in the visual cortex responded to visual stimuli.[6] A highlight of Wiesel’s talk at the Lindau Nobel Laureate Meeting was a short movie of the original 1958 experiment performed in the basement of Johns Hopkins University, which illustrates this principle. In these very “low-tech” experiments performed with David Hubel, Wiesel stimulated individual cells in the visual cortex of cats with small spots of light using a slide projector lying around in the basement and then monitored the window that the cell that they recorded from could “see”.
(00:18:30 - 00:21:14)
The complete video is available here.
In his talk from 1990, Wiesel then goes into detail to explain the Nobel citation accompanying Hubel’s and his award which explicitly mentioned insights into how the ‘image falling on the retina undergoes a step-wise analysis in a system of nerve cells stored in columns.’ The column principle encompasses both ‘orientation columns’ in which cells that respond to light of the same angle are grouped together vertically as well as ‘ocular dominance columns’ that exhibit preference for input from one eye or the other.[6] Later.
(00:16:01 - 00:17:54)
The complete video is available here.
Speech
Although he won his Nobel Prize for work on the synapse, Sir John Eccles chose to devote much of his talk at the 1972 Nobel Laureate Meeting to the work of another laureate, Roger Sperry, who won his award for split-brain research, i.e., for work that revealed how specific brain functions tend to localise to either the left or right hemispheres of the brain, respectively. At the beginning of his lecture, Eccles gives an overview of different brain areas and where specific functions are localised. Of particular relevance for speech are Broca’s area and Wernicke’s area discovered by Pierre Paul and Carl Wernicke, respectively, in the 19th century, and which are usually found in the left hemisphere.
(00:03:16 - 00:04:09)
The complete video is available here.
Sperry’s split-brain research was based on experiments in which he severed the tissue connecting the two brain hemispheres. This was initially undertaken as a treatment for epilepsy as it was thought that it would hinder the two sides of the brain from ‘inciting’ each other during epileptic seizures. Indeed, the technique was highly effective in alleviating epileptic symptoms. Intriguingly, however, that was not all it did. Sperry looked very closely at patients who had undergone this treatment in what Eccles refers to as ‘the most important experiments ever done on human brains’. What did he find?
Sperry provided subjects with visual cues that were selectively perceived either by the right or left eye, which then provide input to the left and right brain hemispheres, respectively. He could then show that the subjects’ capacity to articulate what they had seen was a property harboured only by the left brain. The word ‘pencil’ was shown to the patients in a way that they could only see it with either their left or their right eye. Subjects who were exposed to the word ‘pencil’ in their left visual field could reach out and correctly identify and grab a pencil but could not say what they had done. In contrast, subjects who received the same cue in their right visual field (which provides input to the left hemisphere) had no problems in grabbing the correct object and could also verbally identify the object.[7]
We’ve Come a Long Way, We Have a Long Way Still to Go
Neuroscience and the study of brain function has undoubtedly made tremendous strides over the last 200 years as succinctly summarised here by Erwin Neher:
(00:01:14 - 00:02:44)
The complete video is available here.
In this time, the methodical approach of studying the brain cell by cell – as exemplified by Torsten Wiesel – has borne much fruit: we now understand the basic principles of how the brain goes about its work and also have at least some idea of where specific functions such as movement and speech are localised. What of the future?
Towards the end of his talk in 2018, Edvard Moser addressed the young scientists in the audience directly. His appeal to the next generation of researchers to move beyond the ‘nuts and bolts’ concerns of course primarily his own field of space and time, but his advice is surely relevant for all areas of neuroscience research.
(00:30:18 - 00:30:58)
The complete video is available here.
Further Reading on Neuroscience and the Brain
Past The Beautiful Brain: The Drawings of Ramon y Cajal by Javier DeFelipe and Larry Swanson
Present Navigating cognition: Spatial codes for human thinking by Mellmund et al. in Science
Future Homo Deus: A Brief History of Tomorrow by Yuval Harari
Bibliography
1. Neuroscience. 2nd edition. Purves D, Augustine GJ, Fitzpatrick D, et al., editors. Sunderland (MA): Sinauer Associates; 2001.
2. Animal electricity and the birth of electrophysiology: the legacy of Luigi Galvani. Piccolino M. Brain Research Bulletin, 1998, Jul 15;46(5):381-407
3. Spatial representation in the hippocampal formation: a history. Moser EI, Moser MB, McNaughton BL. Nature Neuroscience, 2017 Oct 26;20(11):1448-1464.
4. Object-vector coding in the medial entorhinal cortex. Høydal ØA, Skytøen ER, Andersson SO, Moser MB, Moser EI. Nature, 2019 Apr;568(7752):400-404.
5. Integrating time from experience in the lateral entorhinal cortex. Tsao A, Sugar J, Lu L, Wang C, Knierim JJ, Moser MB, Moser EI. Nature, 2018 Sep;561(7721):57-62.
6. An introduction to the work of David Hubel and Torsten Wiesel. Kandel ER. J Physiol. 2009 Jun 15;587(Pt 12):2733-41.
7. The "split brain" and Roger Wolcott Sperry (1913-1994). Pearce JMS; FRCP. Rev Neurol (Paris). 2019 Apr;175(4):217-220.