Directed Evolution
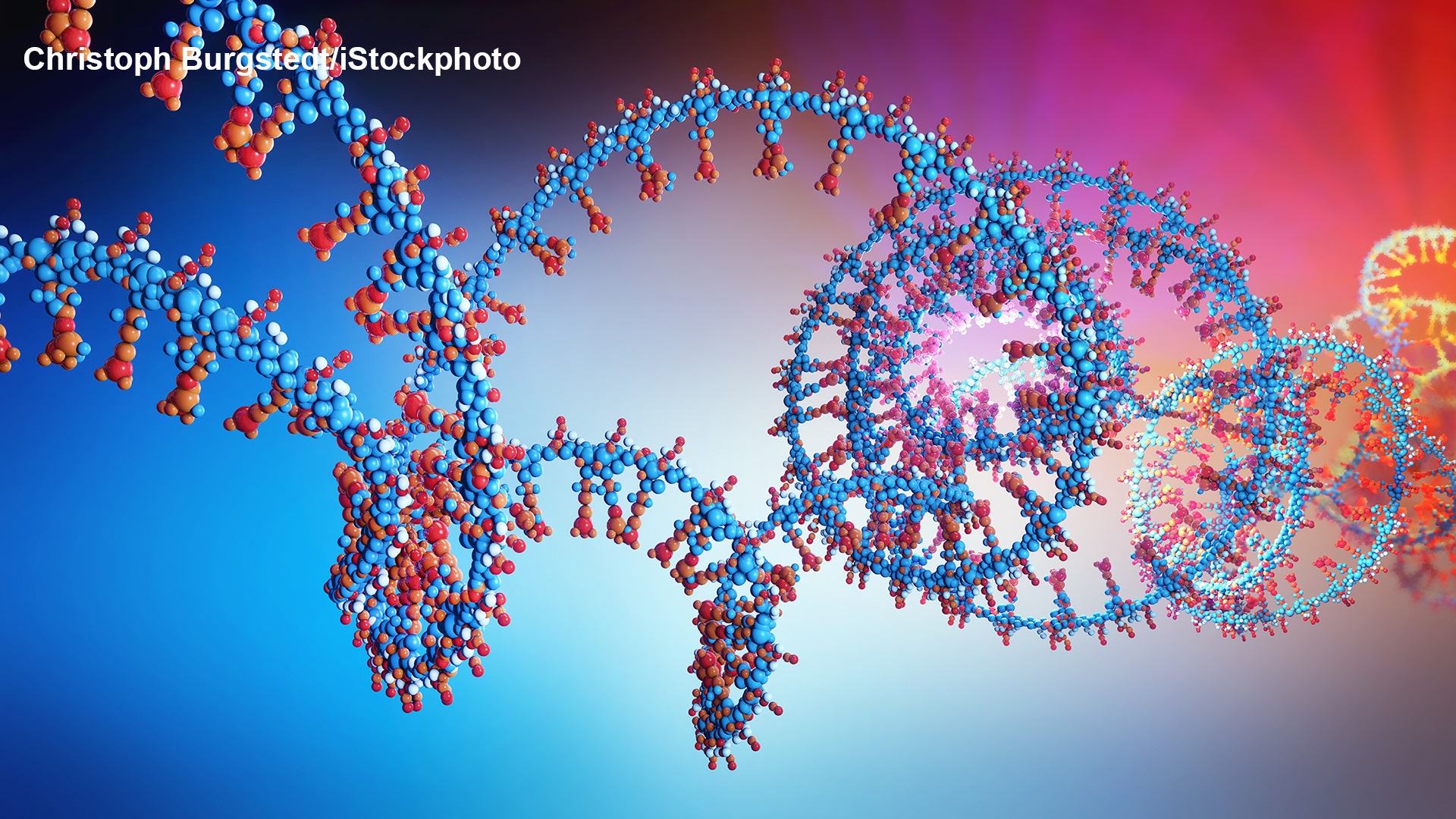
by Hanna Kurlanda-Witek
Selection with a Goal
Carrots weren’t always orange. The vividly-colored vegetable we know today was developed by gardeners in the Netherlands in the 16th century. A keen observer of Dutch paintings of market stalls and kitchen scenes will notice orange carrots in 16th century paintings and paler, yellow carrots in paintings from before that time [1]. The wild mustard plant is a weed-like plant with long stems and small yellow flowers. Over the course of centuries, farmers cultivated different vegetables from the mustard plant by modifying its traits; for instance, suppressing flower development eventually led to the broccoli plant. Kohlrabi, kale, cabbage, cauliflower and brussel sprouts are also descendants of the wild mustard plant [2]. If you look at an equivalent process in animal domestication, it took thousands of years for coarse-haired mountain sheep to become fluffy white bundles that dot green meadows [3]. These examples of plant cultivation and animal breeding are known as artificial selection. Natural selection is the process of adaptation caused by the environment, artificial selection is under human control. The plants and animals with the desired traits – crunchier stalks, uniform hair fibers – were allowed to reproduce, accelerating evolution for human purposes [4]. In his lecture on genetically modified organisms, the Nobel Laureate Sir Richard Roberts noted that with the exception of fish, everything we eat today has been genetically modified in this manner.
(00:04:00 - 00:05:35)
The complete video is available here.
Julia Jansing, a post-doc who participated in the panel on gene editing during the 70th Lindau Nobel Laureate Meeting, further explained problems with public awareness with what she called, “the false dichotomy between natural and modern agriculture”:
(00:46:11 - 00:49:58)
The complete video is available here.
Evolution Under the Microscope
The processes of natural or artificial selection are not linear. It is very much a “trial and error” method, with many dead-ends as a result. In 1986, the Nobel Laureate Konrad Bloch mentioned the term “biological tinkering”, which was first used by another Nobel Laureate, the biologist François Jacob.
(00:17:08 - 00:18:05)
The complete video is available here.
With the development of molecular biology, “tinkering” became achievable at the molecular scale, leading the way to genetic engineering. The new technologies of the last several decades have made it possible to modify the sequence of specific proteins – evolution in a test tube, but with the advantage of gaining a new function or purpose of the protein. Directed evolution uses genetic change and selection to create proteins that may have very useful applications [5]. The first experiments demonstrating that directed evolution was feasible were carried out by Frances Arnold in 1993. Arnold and her team worked on enzymes, which are proteins that catalyze biochemical reactions, or in the words of Arnold, “molecular machines that perform chemistry no human has matched or mastered.” [6] Arnold won half of the Nobel Prize in Chemistry in 2018, “for the directed evolution of enzymes.” In this lecture fragment, she explains why enzymes are so fascinating and how the impressive abilities of evolution can be put to use:
(00:03:14 - 00:05:27)
The complete video is available here.
The Search for the Substance Responsible for Catalysis
“For close to five thousand years we have made use of microbial enzymes to brew beer and leaven bread,” wrote Frances Arnold in her Nobel lecture. However, it wasn’t until the 1830s that the concept of catalysts, substances that can speed up chemical reactions, was perceived. In 1833, Anselme Payen and Jean-François Persoz discovered what they called diastase but is now known as amylase – the enzyme that breaks starch down into sugar [7]. Two years later, the Swedish chemist Jöns Jacob Berzelius came up with the term “catalysis”, but the term “enzyme” was first used in 1877 by Wilhelm Kühne, who had also isolated the pancreatic enzyme trypsin, which facilitates the hydrolysis of proteins [8, 9]. The Nobel Laureate Emil Fischer introduced the lock-and-key model in 1894, where the enzyme (lock) and the substrate (key) have the appropriate geometry to bind together and carry out the reaction. Despite these advances, nobody knew what an enzyme actually was from a biochemical standpoint. The puzzle was solved by James B. Sumner in 1926, who crystallized urease and demonstrated that it was a protein. “All enzymes are proteins but not all proteins are enzymes”, said Sumner in his Nobel lecture, “Chemical Nature of Enzymes” [10]. Fischer’s lock-and-key theory was altered by the time Sumner received his Nobel Prize in 1946. William Lipscomb explained in Lindau in 1980, that “enzymes are not just inert templates on which the reaction occurs (...), enzymes do chemistry.”
(00:30:43 - 00:31:44)
The complete video is available here.
Lipscomb concluded his lecture by saying that, “Enzyme-catalyzed reactions proceed very rapidly compared with most of the models. Enzymes do indeed use ordinary chemical mechanisms, but they use the specific binding interactions to help speed up reactions (...).”
The regulatory mechanisms of enzymes, in this case proteases (enzymes that catalyze the degradation of proteins), were explained by Nobel Laureate Robert Huber in Lindau in 2019. Huber presented the diversity of these enzymes and the clever control methods they employ, which prevent them from destroying our own bodies.
(00:27:07 - 00:32:13)
The complete video is available here.
“I strongly believe that, through the products of organic synthesis, it will be possible to gain influence over the development of organisms and to produce changes that surpass all that can be achieved by conventional breeding.” – Emil Fischer, 1917, Nobel Prize in Chemistry 1902 [11]
In the 1940s and 1950s, there were numerous discoveries of individual proteins, their structures and mechanisms. But at around the same time, a new molecule arrived at the scene; once the structure of DNA was revealed in 1953, the fields of protein and DNA chemistry developed in parallel [12]. Tellingly, the Nobel Prize in Chemistry in 1958 was awarded to Frederick Sanger for deciphering the amino acid sequence in the hormone insulin, the first protein to be sequenced, and in the same year, half of the Nobel Prize in Physiology or Medicine went to George W. Beadle and Edward L. Tatum for proposing the “one gene – one enzyme” theory. They carried out experiments where the Neurospora fungi was treated with x-rays, creating mutants that were unable to synthesize vitamin B6. “Inability to synthesize vitamin B6 is apparently differentiated by a single gene from the ability of the organism to elaborate this essential growth substance,” concluded Beadle and Tatum in their research paper; consequently, changing a specific gene determines whether an enzyme is produced by an organism or not [13]. Astonishingly, these experiments on genes were carried out in 1941, a few years before it was established that DNA was the carrier of genetic information.
The biochemistry of nucleic acids spurred the interest of enzymologists and biochemists, as described here by Michael Smith in Lindau in 1995. Smith’s mentor and 1968 Nobel Laureate H. Gobind Khorana, used enzymes to build short DNA molecules, a discovery that led to the first synthesis of a gene [11]. Further discoveries, namely, of restriction enzymes, which can cut out specific sections of DNA; DNA-ligases, responsible for joining or repairing DNA fragments; or DNA polymerases, which duplicate a cell’s DNA before replication, changed not only the perception of enzymology, but formed the basis of recombinant technology and genetic engineering.
The extract of Michael Smith' lecture “Synthetic DNA and Biology” (1995), that is relevant for this paragraph, goes from 6:00 – 7:11. The complete audio of the lecture is available here.
It now became possible to insert DNA from one organism to another, for example to produce human proteins in bacterial cells. This had an enormous impact on the production of pharmaceuticals. The first recombinant protein to be used commercially was insulin, grown in E. coli cells. Since 1982, this technology has been used to produce human insulin, widely replacing animal-sourced insulin from pigs and cows [12]. The growing market of the industrial application of enzymes was mentioned by Nobel Laureate Christian B. Anfinsen in 1989:
(00:08:08 - 00:09:25)
The complete video is available here.
The Path Towards Directed Evolution
The widespread synthesis and use of existing enzymes for various purposes is in itself remarkable but finding new proteins as a result of artificial mutations in genetic material significantly expands the possibilities for discovery.
Werner Arber, who was awarded the Nobel Prize in Physiology or Medicine in 1978 for the discovery of restriction enzymes, gave an overview of the types of mutations during a lecture in Lindau in 2009:
(00:10:40 - 00:15:44)
The complete video is available here.
In 1978, Michael Smith and colleagues published a paper on site-directed mutagenesis, a novel technique, where short DNA molecules (oligonucleotides) are used to create mutations in gene sequences. The mutation can be generated by adding, deleting or substituting nucleotides, the building blocks of DNA [14]. According to Smith, who won the Nobel Prize in Chemistry in 1993, the potential of site-directed mutagenesis was obvious from the beginning, “however, we could not have anticipated the explosion of gene isolations, the improvements in DNA sequence determination methodology and the advances in the chemistry of nucleic acid synthesis that have occurred since 1978. This has resulted in an amazing increase in the use of site-directed mutagenesis as an analytical tool in biochemistry and biology.” [11] The technique formed the foundation for protein engineering – the ability to modify the nucleotides of a specific gene and observe the effects on the resulting protein.
Smith’s co-recipient of the Nobel Prize was Kary B. Mullis, who invented polymerase chain reaction (PCR), a method of creating significant amounts of copies of a small piece of DNA. Heat causes the double helix of a DNA fragment to disconnect. A heat-resistant type of DNA polymerase, isolated from the bacteria Thermus aquaticus, is used to build the DNA fragment of interest, and the cycle is repeated again and again, producing large numbers of copies, even millions of copies in hours, from a single strand of DNA [12, 15].
Turning up the Crank of Artificial Selection
Site-directed mutagenesis and PCR set the stage for directed evolution. These new technologies made it achievable to create extensive libraries of mutants and to screen the produced proteins for features chosen by the user. But there was one important development that changed the perspective of how experiments were done. During the 40th Lindau Nobel Laureate Meeting in 1990, Hamilton O. Smith, who won the Nobel Prize in Physiology or Medicine in 1978, explained the transformation caused by the advent of the personal computer; “The development of the personal computer ... puts within the hands of every scientist the ability to test new ideas.”
(00:09:15 - 00:10:43)
The complete video is available here.
In the 1980s, Frances Arnold implemented a process of mutating the protease subtilisin E, which can break down the milk protein casein, to carry out its activity in an organic solvent (dimethylformamide, or DMF) instead of water. The idea was that enzymes could be optimized to carry out their functions in non-natural conditions. The mutated genes were inserted in bacteria, which created a library of thousands of subtilisin variants. Screening identified the rare variant that hydrolyzed casein most effectively in a low-concentration solution of DMF. This variant was selected for additional mutations, and the cycle was repeated, with rising concentrations of DMF. Finally, this speedy evolution resulted in a variant of subtilisin that could function in 60% DMF, compared to the original subtilisin [6, 16]. Arnold explained how to re-optimize an enzyme for a new job in her lecture during the Online Science Days in 2020:
(00:09:06 - 00:11:21)
The complete video is available here.
Interestingly, the concept of accelerating enzyme efficiency, mimicking natural evolution, was not new. In 1980, the chemist and Nobel Laureate Sir John Cornforth presented his work on changing the chemical structure of enzymes to the audience at Lindau.
(00:00:25 - 00:02:59)
The complete video is available here.
“The objective was to develop a stable, easily prepared and efficient catalyst which would work at room temperature in an aqueous environment and which might have commercial application,” wrote Rupert Purchase and James R. Hanson in a 2015 article describing Cornforth’s work in synthetic chemistry [17]. The challenge was to accelerate the properties nature could have devised, but in a laboratory at a much shorter time scale. “I do not have a thousand million years to complete this work!” said Cornforth, and this was echoed in Arnold’s 2018 Nobel lecture. But Cornforth focused solely on analytical chemistry, relying on laborious tinkering with chemical structure to get results. The power behind directed evolution lies in its multifaceted approach, combining methods from analytical chemistry, molecular biology and microbiology [14].
Scratching the Surface of Possibilities for New Enzymes
The methods devised by Arnold in the 1990s have been expanded by newer technologies and importantly, directed evolution has made many industrial applications feasible. “We have just scratched the surface of what nature can do,” said Arnold, and utilizing nature’s strategies in evolution is already fulfilling the principles of green chemistry, such as preventing waste, using less hazardous materials in chemical synthesis, or designing products with energy efficiency in mind [12]. During her online lecture in 2020, Arnold described how pheromones developed by directed evolution are successfully used as a biopesticide to curb damage to corn crops by the fall armyworm:
(00:15:20 - 00:15:56)
The complete video is available here.
There are still many challenges ahead for directed evolution. It is difficult to predict enzyme function; we can mutate the gene, design protein structure, but how can we be sure of the function this enzyme will have, if at all? Protein engineering still requires considerable amounts of time and money before a solution to a real-world problem comes to fruition [12]. But the appealing perspective of custom-made enzymes, upgraded for the benefit of a cleaner environment, more efficient products and therapeutics will make it all worth it in the long term. Directed evolution is here to stay.
Footnotes
[2] https://evolution.berkeley.edu/evolibrary/article/evo_30
[3] Jackson, N., Maddocks, I.G., Watts, J.E., Scobie, D., Mason, R.S., Gordon-Thomas, C., Stockwell, S., and Moore, G.P.M. (2020). Evolution of the sheep coat: the impact of domestication on its structure and development. Genetics Research 104, e4.
[4] Gregory, T.R. (2009). Artificial selection and Domestication: Modern Lessons from Darwin’s Enduring Analogy. Evolution: Education and Outreach 2, pp. 5-27.
[5] https://www.nobelprize.org/prizes/chemistry/2018/press-release/
[6] https://www.nobelprize.org/uploads/2018/10/arnold-lecture.pdf
[8] https://www.nobelprize.org/prizes/chemistry/1989/8985-the-history-of-biocatalysis/
[9] https://www.oxfordreference.com/view/10.1093/oi/authority.20110803100044728
[10] https://www.nobelprize.org/uploads/2018/06/sumner-lecture.pdf
[11] https://www.nobelprize.org/uploads/2018/06/smith-lecture-1.pdf
[12] Heckmann, C.M. and Paradisi, F. (2020) Looking Back: A Short History of the Discovery of Enzymes and How They Became Powerful Chemical Tools. Chem Cat Chem 12, pp. 6082 – 6102.
[13] Beadle, G.W. and Tatum, E.L. (1941). Genetic Control of Biochemical Reactions in Neurospora. Proc Natl Acad Sci USA 27(11), pp. 499-506.
[14] Enqvist, M.K.M. and Rabe, K.S. (2019). Applications of Protein Engineering and Directed Evolution in Plant Research. Plant Physiology 179, pp. 907 – 917.
[15] https://www.nobelprize.org/prizes/chemistry/1993/mullis/facts/
[16] https://www.nobelprize.org/uploads/2018/10/popular-chemistryprize2018.pdf
[17] Purchase R. and Hanson, J.R. (2015). Sir John Cornforth AC CBE FRS: his synthetic work. Science Progress 98(3), 219 – 229.