Photosynthesis
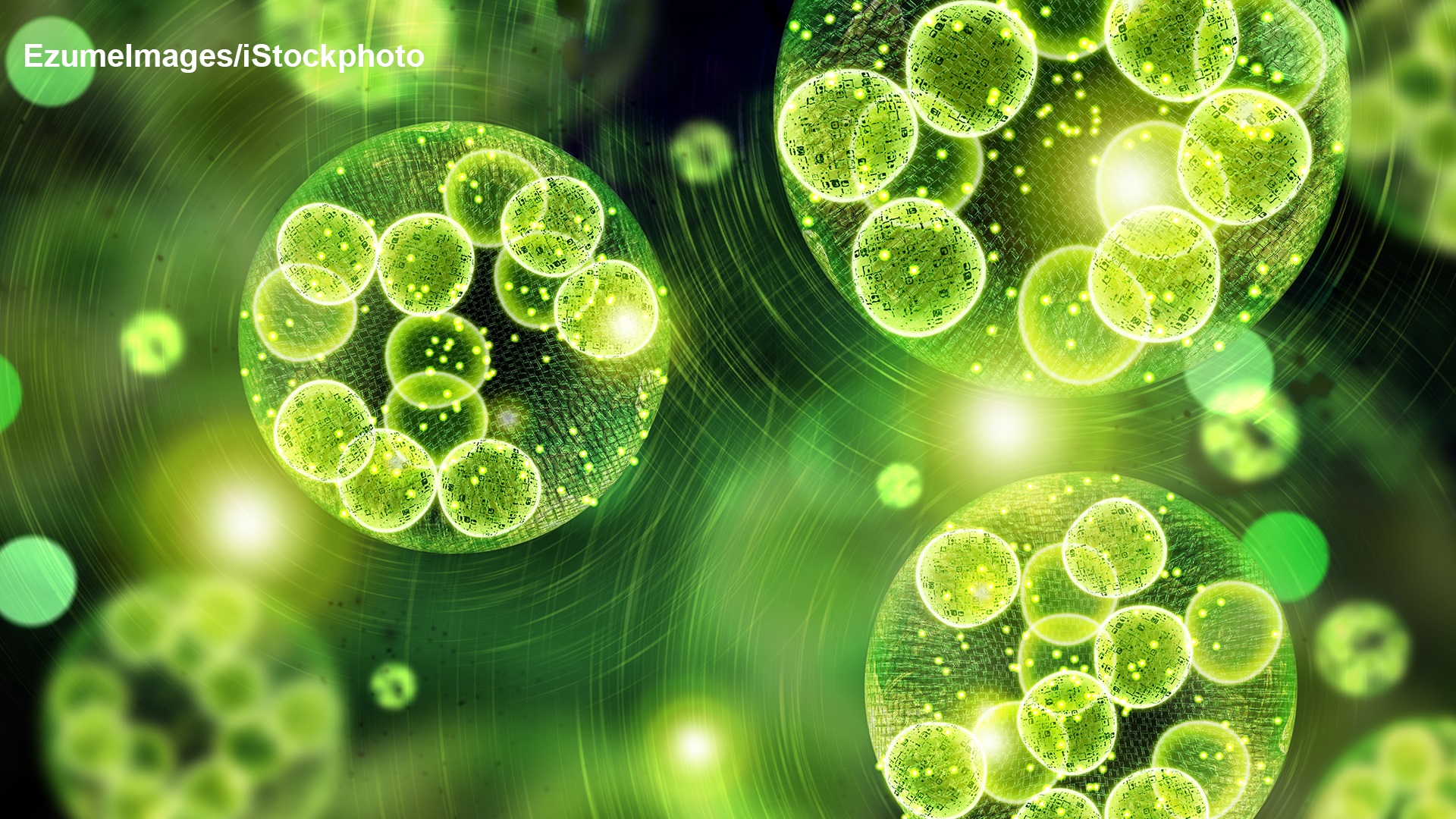
by Meeri Kim
Photosynthesis
Earth is a planet that thrives and teems with life of incredible diversity, from plants of all shapes and sizes to animals that span a vast spectrum of complexity. The engine that powers the majority of life in the biosphere is photosynthesis[1]. This crucial process allows plants, algae, and some single-celled organisms to support themselves by converting light energy into chemical energy, which is used to synthesise organic compounds. In addition, it indirectly sustains most of the life in the biosphere by providing organic matter, food and oxygen.
The following clip from a Mini Lecture on photosynthesis describes why no other chemical process is as crucial for the existence of life.
(00:00:15 - 00:01:20)
The complete video is available here.
Sir John E. Walker covered the basics of photosynthesis and its importance to life at the beginning of his Lindau lecture in 2013. He received the 1997 Nobel Prize in Chemistry for elucidating the structure of adenosine triphosphate (ATP) synthase, an enzyme which assists in the building of ATP molecules that both plants and animals use to store energy[2].
(00:01:22 - 00:02:37)
The complete video is available here.
The Power of Plants
As early as the late 1700s, scientists began to notice the mysterious power of green plants. English chemist Joseph Priestly knew that a mouse placed into a sealed, glass container would quickly die, and the resulting air was rendered “noxious.” But interestingly, when he put a plant inside along with the mouse, the animal survived. Priestly concluded that plants “tend to keep the atmosphere sweet and wholesome.”[3]
In 1779, Dutch physician Jan Ingenhousz published the results of a study that involved over 500 experiments on plants[4]. He put the pieces together that light was a necessary ingredient for a plant to “clean” the air, and only the green parts of the plant actually perform this job.
The chloroplast, an organelle within the cells of plants and green algae packed with chlorophyll, serves as the hub where photosynthesis takes place. It was first described as “a grain of chlorophyll” in 1837, but more than a century of research revealed the breathtaking complexity of photosynthetic structures and reactions. In his 2017 Lindau lecture, Johann Deisenhofer describes what scientists now know about the chloroplast and its machinery. He won the 1988 Nobel Prize in Chemistry along with Robert Huber and Hartmut Michel for unveiling the three-dimensional structure of a photosynthetic reaction centre.
(00:03:40 - 00:06:43)
The complete video is available here.
Early Nobel Prize-Winning Work in Photosynthesis
Several scientists have received the Nobel Prize for their discoveries related to photosynthesis, beginning with Richard Martin Willstätter in 1915[5]. The German organic chemist investigated plant pigments — including chlorophyll, the green pigment vital to photosynthesis — in the two years before World War I. He eventually determined the chemical composition of chlorophyll by extracting the photosynthetic cell and breaking it down into smaller components[6].
Fifteen years later, Hans Fischer got the Nobel Prize in Chemistry mainly for his studies on hemoglobin, the substance that gives blood its red colour, but also for his work on chlorophyll[7]. Two more were given to Paul Karrer (Chemistry, 1937) and Richard Kuhn (Chemistry, 1938) for their research into other plant pigments.
Around this time, Melvin Calvin had just begun his academic career at the University of California, Berkeley as a member of the faculty. The institution had a unique set of tools available for Calvin and his colleagues, including radioactive isotopes and chromatography, that would allow them to develop four different photosynthesis models between 1948 and 1954[8]. The most recent one is still recognised today with slight modification.
Using radiolabelled carbon dioxide, he was able to identify the chemical reactions in the intermediate stages of photosynthesis, receiving the 1961 Nobel Prize in Chemistry for his efforts. In particular, he discovered the dark reaction or Calvin cycle, which doesn’t require light to convert carbon dioxide into carbohydrates[9].
While the first stage of photosynthesis uses energy from light, the second light-independent stage converts carbon dioxide and water into organic molecules such as glucose. The Calvin cycle has three main steps: carbon fixation, reduction, and regeneration. First, carbon dioxide is fixed from an inorganic form to organic molecules with the help of a special enzyme. Next, energy carriers that result from the first stage of photosynthesis convert the product of carbon fixation into a chemical called glyceraldehyde 3-phosphate (G3P), of which six molecules are created. A single molecule of G3P leaves the Calvin cycle to contribute to the formation of other compounds needed by the plant, including glucose and starch, while the remaining five G3P molecules remain in the cycle for carbon fixation in the next cycle[10].
Structural Biology Meets Photosynthesis
Photosynthesis was once again thrust into the world’s scientific spotlight in 1988, when three biochemists were awarded the Nobel Prize in Chemistry for their work on “the most important chemical reaction on earth”[11]. Their subject of study was the purple bacterium Rhodopseudomonas viridis, an organism that performs a simpler form of photosynthesis that does not generate oxygen. However, like green plants, it possesses a photosynthetic reaction centre made up of proteins, pigments, and other chemicals that together execute energy conversion.
Shortly after receiving his doctorate, Hartmut Michel achieved what was once regarded as an impossible feat. He crystallised a membrane-bound protein complex — the aforementioned photosynthetic reaction centre extracted from R. viridis — into a form pure enough for X-ray diffraction techniques[12]. Along with Johann Deisenhofer and Robert Huber, Michel employed X-ray crystallography to reveal the exact arrangement of the more than 10,000 atoms that make up the photosynthetic reaction centre[13]. The 1985 discovery was also noteworthy as being the first 3D crystal structure of a membrane protein complex.
Only three short years later, the trio won the Nobel Prize for greatly contributing to the world’s understanding of photosynthesis and membrane proteins in general. In this clip from his 2014 Lindau lecture, Michel describes how the phrase “it is impossible to crystallise membrane proteins” once appeared in the most commonly used biochemistry textbook of its time — and how he refused to believe it.
(00:05:15 - 00:07:53)
The complete video is available here.
The Energy Currency of Life
The most recent Nobel Prize related to photosynthesis (Paul D. Boyer and John E. Walker, 1997) covers an even broader process which also applies to cellular respiration in mitochondria: the formation of ATP[14]. The molecule represents the energy currency of life, as all living organisms — from bacteria and fungi to plants and animals — use ATP for chemical energy storage and transfer.
The enzyme ATP synthase sits in the membrane of chloroplasts and catalyses the synthesis of ATP from adenosine diphosphate (ADP) and phosphate[15]. The driving force behind this reaction is the energy stored in the proton gradient, or the difference in hydrogen ion concentration between the inside and outside of the membrane. Essentially, a high energy electron is passed along an electron transport chain, which forces hydrogen ions out of the chloroplast[16]. The resulting gradient drives hydrogen ions back inside through ATP synthase and kicks off the reaction that creates ATP.
The following Mini Lecture outlines how photons from sunlight excite electrons in chlorophyll to an energetically higher state and then pass through a series of transport steps.
(00:02:54 - 00:03:45)
The complete video is available here.
In 1974, American biochemist Paul D. Boyer presented a theory on how ATP synthase worked, called the binding-change hypothesis. It described in detail how the enzyme catalyses the synthesis of ATP from ADP and phosphate [17]. Over two decades later, the theory was experimentally validated when British chemist John E. Walker used X-ray crystallography to determine the enzyme’s structure [18]. Boyer and Walker each received one-quarter of the 1997 Nobel Prize in Chemistry for their respective work on ATP synthase.
In the following clip from his 2014 Lindau lecture, Walker talks about the advances in imaging that have elucidated ATP synthase in even further atomic detail, and what differences are found in the molecule between mitochondria and bacteria/chloroplasts.
(00:17:42 - 00:21:26)
The complete video is available here.
The Most Important Chemical Reaction on Earth
Even Nobel Laureates who had received their prize for work in a different field delved into the mysteries of photosynthesis in an attempt to decipher the fundamental process. Otto Warburg, a German biochemist who was awarded the 1931 Nobel Prize in Physiology or Medicine, published research on photosynthesis for fifty years. While best known for his discoveries on respiratory enzymes, Warburg also famously held fast to his outdated concepts of photosynthesis in the face of overwhelming evidence to the contrary [19].
Most notably, he experimentally determined the maximum quantum yield of photosynthesis, or the minimum number of light quanta required to output one molecule of oxygen, to be a value of 3 or 4. One of his former students, Robert Emerson, consistently obtained a value of about 10. Until his death, Warburg stubbornly insisted his finding was correct. Ultimately, additional research into the matter by several other groups favoured Emerson’s result.
Warburg discussed photosynthesis during his lecture at the very first Lindau Nobel Laureate Meeting in 1951, as well as in subsequent meetings in 1954 and 1963. The following is a clip from his second Lindau lecture, which covered the controversial issue of quantum yield — and serves as a lesson to young scientists to listen even to Laureates with a critical ear.
(00:07:30 - 00:09:54)
The complete video is available here.
For none-German speaking persons: Please turn on the subtitles using the speech bubble at the lower right corner.
Both James Franck (Physics, 1925) and George Porter (Chemistry, 1967) also dabbled in photosynthesis. Franck spent most of his time and energy on constructing an overall theory of photosynthesis consistent with major observations [20]. However, his theories turned out to be inconsistent with experiments or later empirical results. Porter used ultrashort pulses of light to investigate the first few femtoseconds of the process[21], and also studied what he called in vitro photosynthesis, or systems which imitate parts of the natural photosynthetic process. He felt that mimicking the reaction in the lab could elucidate unknown parts of the process, as well as provide a sustainable energy resource to replace fossil fuels.
Now that the complex mechanisms of photosynthesis have been elucidated, what is next for scientific research in this field? Artificial photosynthesis, which encompasses technology that stores the sun’s energy in chemical bonds, is an area of highly active research. The field looks to natural photosynthesis for inspiration as it attempts to find a more sustainable way to store and distribute energy. The most popular approach is using photocatalytic water splitting to convert water into hydrogen as a solar fuel and oxygen[22].
In addition, there is considerable interest in manipulating the process to solve the world's environmental issues, such as climate change and food scarcity. If the efficiency of plant photosynthesis were pushed far beyond the current five percent sunlight-to-biomass energy conversion rate [23], crops could more easily feed a growing world population, and carbon dioxide concentrations in the atmosphere could decrease. Researchers are currently testing out genetic engineering and more aggressive techniques of synthetic biology to improve upon inefficiencies in the natural process. For instance, scientists working on the Realising Increased Photosynthetic Efficiency (RIPE) project, an international consortium based at the University of Illinois at Urbana-Champaign, demonstrated in 2016 that increasing the expression levels of three genes involved in processing light improved tobacco crop productivity by 15 percent[24].
In his 2012 Lindau lecture, Michel describes three potential approaches to increasing the conversion efficiency of photosynthesis – but notes that water availability will be a crucial factor since it is a limiting factor in biomass yield.
(00:17:46 - 00:19:26)
The complete video is available here.
Lastly, photosynthesis allows green plants to store large amounts of carbon in above- and below-ground biomass. Their fundamentally important role as carbon sinks has led researchers to consider ways to increase the amount of atmospheric carbon dioxide they capture as a way to offset greenhouse gas emissions. As an example, some experts believe that genetic engineering approaches in plant breeding can enhance the capacity for plants to serve as carbon sinks, also known as phytosequestration[25].
Related Videos
Sir John E. Walker 2017: "The ATP Synthase in Cellular Life and Death"
Johann Deisenhofer 2016: "Photosynthetic Light Reactions, Revisited"
Sir John E. Walker 2013: "The Fuel of Life"
Paul Boyer 2002: "The Oxygen We Breathe - Friend and Foe"
Hartmut Michel 2012: "Photosynthesis, Biomass, Biofuels: Conversion Efficiencies and Consequences"
Johann Deisenhofer 1989: "The Three-Dimensional Structure of a Membrane Protein"
Hartmut Michel 1989: "Structure and function of a biological light energy converter"
Bibliography
[1] Lambers, H. and Bassham, J. A. (2018) Photosynthesis. In Encyclopaedia Britannica Online. Retrieved from http://www.britannica.com
[2] Nobel Prize in Chemistry 2017: Press Release
[3] Priestly, J. (1772) XIX. Observations on different kinds of air. Philosophical Transactions of the Royal Society of London. Volume 62.
[4] Ingenhousz, J. (1779) Experiments upon Vegetables, Discovering Their Great Power of Purifying the Common Air in Sunshine, and of Injuring It in the Shade and at Night.
[5] Nobel Prize in Chemistry 1915: Summary
[6] Willstätter, R. and Stoll, A. (1913) Untersuchungen über Chlorophyll. Published by Julius Springer, Berlin.
[7] Nobel Laureate Hans Fischer: Facts
[8] Hahn, A. M. (2016) Can a History of Photosynthesis be Grand? A Review of Explaining Photosynthesis: Models of Biochemical Mechanisms, 1840–1960, by Kärin Nickelsen, Springer, 2015. Philosophy and Theory in Biology, Vol. 8
[9] Calvin, M. (1960) The path of carbon in photosynthesis. Die CO2-Assimilation/The Assimilation of Carbon Dioxide, 884-922
[10] Raines, C.A. (2003) The Calvin cycle revisited. Photosynthesis Research. Vol. 75, 1-10
[11] Nobel Prize in Chemistry 1988: Press Release
[12] Deisenhofer, J. and Michel, H. (1989) Nobel lecture. The photosynthetic reaction centre from the purple bacterium Rhodopseudomonas viridis. The EMBO Journal. Vol. 8, 2149–2170
[13] Deisenhofer, J., Epp, O., Miki, K., Huber, R. and Michel, H. (1985) Structure of the protein subunits in the photosynthetic reaction centre of Rhodopseudomonas viridis at 3Å resolution. Nature. Vol. 318, 618–624
[14] Nobel Prize in Chemistry 1988: Press Release
[15] The Editors of Encyclopaedia Britannica. (2019) Adenosine triphosphate. In Encyclopaedia Britannica Online. Retrieved from http://www.britannica.com
[16] Electron Transport in Photosynthesis
[17] Boyer, P.D. (1993) The binding change mechanism for ATP synthase — Some probabilities and possibilities. Biochimica et Biophysica Acta (BBA) - Bioenergetics. Vol. 1140, 215-250
[18] Abrahams, J.P., Leslie, A.G.W., Lutter, R. and Walker, J.E. (1994) Structure at 2.8 Â resolution of F1-ATPase from bovine heart mitochondria. Nature. Vol. 370, 621-628
[19] Hill, J. F. and Govindjee. (2014) The controversy over the minimum quantum requirement for oxygen evolution. Photosynthesis Research. Vol. 122, 97-112
[20] Rosenberg, J. L. (2004) The contributions of James Franck to photosynthesis research: a tribute. Photosynthesis Research. Vol. 80, 71-76
[21] Nobel Laureate George Porter: Biographie
[22] Tachibana, Y., Vayssieres, L., and Durrant, J. Artificial photosynthesis for solar water-splitting. Nature Photonics. Vol. 6, 511-518
[23] Blankenship, R.E. et al. (2011) Comparing Photosynthetic and Photovoltaic Efficiencies and Recognizing the Potential for Improvement. Science. Vol. 332, 805-809
[24] Kromdijk, J. et al. (2016) Improving photosynthesis and crop productivity by accelerating recovery from photoprotection. Science. Vol. 354, 857-861
[25] Jansson, C. et al. (2010) Phytosequestration: Carbon Biosequestration by Plants and the Prospects of Genetic Engineering. BioScience. Vol. 60, 685-696