Prof. Dr. Maurice Hugh Frederick Wilkins > Research Profile
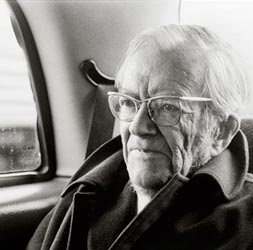
by Luisa Bonolis
Maurice Hugh Frederick Wilkins
Nobel Prize in Physiology or Medicine 1962
From Radar Research to Biophysics
Like Ernst Rutherford, Maurice Wilkins came from New Zealand. He was born in 1916 from Anglo-Irish parents in the South Island mountain town of Pongoroa, near Wellington. His father, Edgar Wilkins, was a doctor with the School Medical Service, with a strong interest in public health. In 1923, the family returned to the UK and lived in Birmingham, where Wilkins soon showed an early interest in astronomy and optics building his own telescopes and microscopes. In 1935, he won a scholarship and was admitted to St John’s College, in Cambridge, where he obtained a degree in Physics in 1938. He then followed his former tutor Mark Oliphant, who had been Ernest Rutherford's deputy of research at Cambridge, and had now been appointed to the chair of Physics at the University of Birmingham. Wilkins became a Ph.D. student under John Randall, who had previously worked at the General Electric Company, a brilliant physicist, who had been recruited by Oliphant to revitalise the department. After the outbreak of World War II, nearly the entire Department of Physics was involved in defence research on radar. At the time, limited transmitter output was the greatest single obstacle in the development of radar. In 1939, working with Harry Boot, John Randall developed a working prototype of cavity magnetron, which was a thousand times more powerful than any other microwave transmitter at the time and became the all-important source of centimetric radar waves and one of the keys to the Allied victory in the Second World War.
Wilkins completed his Ph.D. in 1940 with a dissertation on luminescence and the movement of electrons in crystals. He was then recruited into the Ministry of Home Security and Aircraft Production to work on the improvement of cathode-ray tube screens for radar. The technology he developed contributed to wartime efforts and is still used in modern radar. He later worked under Oliphant, studying isotope separation, a crucial process for the creation of uranium-based nuclear weapons. In 1944, together with other British scientists, he moved to the United States, following Oliphant to the University of California, at Berkeley, to help fractionate uranium at Ernest Lawrence's cyclotron laboratory. They all worked as part of the Manhattan project, the American wartime initiative that led to the completion, and use, of the two nuclear bombs dropped on the Japanese towns Hiroshima and Nagasaki in August 1945. After these dramatic events, Wilkins became increasingly concerned about the ethical implications of nuclear weapons, whose catastrophic possibilities he had not fully considered during his time at Berkeley, under the pressure of war efforts aimed at gaining the technological upper hand over Hitler's Germany. It was during this time that he read Erwin Schrödinger's book, What is Life? The physical aspects of the living cell, which had recently been published. Schrödinger proposed that through quantum physics it was possible to understand biological growth and the nature of life itself. Like many young scientists of his generation, Wilkins was struck by the concept of a highly complex molecular structure that controlled living processes. Fascinated by the novelty of these new concepts involving crossover methodologies of previously unrelated disciplines, he decided to turn away from nuclear physics to pursue biophysics, a branch of science with more positive applications. He was not alone. At that time many leading physicists believed that physics would contribute significantly to biology.
The Secret of Life
After returning from California, Wilkins joined Randall's research group at the University of Birmingham. In 1946 the unit moved to King's College, London, where Randall was appointed full professor of physics. Randall was starting a new project exploring the links between physics and biology. With funding from both the Rockefeller foundation and the Medical Research Council, Randall built up a Biophysics Unit, an unusual laboratory where biologists, biochemists and others worked with the physicists.
Randall appointed Wilkins Assistant Director of the newly formed Medical Research Council Biophysics Research Unit at King's College, and Wilkins cast about for a research subject within the complex structure of the cell. He initially took over ultraviolet microscope studies of the quantities of nucleic acids in cells. The nucleic acids had been discovered in the late 1860s by the Swiss biochemist Friedrich Miescher. At that same time the Czech monk Gregor Mendel had been able to show that certain traits in the peas, such as their shape or colour, were inherited in different packages. These packages are what were later called genes. For a long time the connection between nucleic acid and genes was not known. During the early decades of the twentieth century, biochemists had gradually elucidated their chemical structure, and in 1944, Oswald Avery had shown that genes consist of DNA, one of the nucleic acids. But at that time most biochemists had doubts whether something as simple as what DNA was thought to be - repetitions of the four nucleotide bases - could be the genetic substance. More complex molecules like proteins - chromosomal proteins - were thought to be more likely candidates. However, between 1946 and 1950 many lines of evidence confirmed that the genetic substance was DNA, not protein or nucleoprotein.
Wilkins used ultraviolet microscopes to study proteins, tobacco mosaic virus, vitamin B12 and soon narrowed his focus to DNA. Samples of high-quality DNA had been brought to London by the Austrian scientist Rudolf Signer, at a meeting on nucleic acids held in May 1950. At that time it was known that the nucleic acids occur in two forms: DNA and ribonucleic acid (RNA). Both RNA and DNA are made of five-carbon sugar molecules (ribose or deoxyribose), phosphate, and four nitrogen-containing bases - adenine, thymine (replaced by uracil in RNA), guanine, and cytosine. While preparing the samples for X-raying, Wilkins realised that every time he was manipulating the moist DNA gel under a microscope with a glass rod, a thin and almost invisible fibre of DNA was drawn out like a filament of spider's web. As he recalled in his Nobel lecture, “The perfection and uniformity of the fibres suggested that the molecules in them were regularly arranged.” Examination under polarised light showed them to be well ordered. Wilkins came to the conclusion that the detailed molecular structure of DNA had to be determined. However, for studying such a high-molecular mass polymer, he needed much smaller probe wavelengths than those of his usual microscopes. Wilkins immediately thought the fibres might be excellent objects to study by X-ray diffraction analysis and took them to Raymond Gosling, a graduate student who had their X-ray equipment in the laboratory and who was using it to obtain diffraction photographs from heads of ram spermatozoa.
In 1912, the German physicist Max von Laue had put forward the idea that atomic sheets were about the right size to act like the slits in a diffraction grating for X-rays, as the space between them was of the same order of magnitude as their wavelength, much shorter than the size of visible light. His prediction was soon confirmed by firing a beam of X-rays at a crystal of zinc sulphide and capturing the scatter on a photographic plate. When the plate was developed, there was a circular pattern of light and dark spots -- a diffraction pattern. This proved that X-rays behaved like waves, settling a controversy that had lasted the 17 years since their discovery. Max von Laue was awarded the 1914 Nobel Prize in Physics “for his discovery of the diffraction of X-rays by crystals”. Once they had a beam of a specific wavelength, researchers could use X-rays to study the spacing of the crystal gratings: X-ray crystallography became the first probe for “seeing” the three-dimensional structure of matter at the atomic level. Henry and Lawrence Bragg, father and son, immediately pioneered the new technique, which involves a lot of mathematical analysis, and were able to determine the internal architecture of some crystalline solids, and thus to explain their properties.
The Braggs shared the 1915 Nobel Prize in physics “for their services in the analysis of crystal structure by means of X-rays”. At 25, Lawrence Bragg is still the youngest ever recipient of the Nobel Prize. In 1919 he succeeded Ernest Rutherford as chair of physics at the University of Manchester and in 1938 he again replaced Rutherford, this time as Cavendish professor at the University of Cambridge. Since the dawn of modern crystallography with X-ray diffraction experiments on crystals, the discipline has informed almost every branch of science, providing a means to understand the structure of complex molecules and materials. After the war, Bragg encouraged his protégés Max Perutz and John Kendrew in their work on the X-ray crystallographic determination of the proteins haemoglobin and myoglobin, a path that would eventually lead them to the 1962 Nobel Prize for Chemistry.
Randall, who had been a Ph.D. student of Lawrence Bragg’s, organised studies into biophysics at King's College also with a view to applying X-ray crystallography to biology. Wilkins had had some training in X-ray crystallography during his studies at Cambridge, where the field was under the influence of John Bernal, then at the Cavendish Laboratory. In 1934, Bernal, who was a pioneer in the use of X-ray crystallography in molecular biology, had taken the first X-ray photographs of hydrated protein crystals with the young Dorothy Hodgkin, who became one of the main founders of protein crystallography. At that time, Max Perutz arrived as a student from Vienna and started to work on haemoglobin. Both Hodgkin and Perutz would be awarded the Nobel Prize in Chemistry during the 1960s for solving the structure of important biochemical molecules. The great British tradition of X-ray crystallography initiated by Henry and Lawrence Bragg was now flourishing and after the war financial support allowed the establishment of specific laboratories for the research into the molecular structure of biological systems. The Molecular Biology Unit, for example, at Cavendish Laboratory in Cambridge attracted researchers who felt that the new field of molecular biology had great promise. Among them were Francis Crick and James Watson.
The Race for DNA Three-Dimensional Structure
Wilkins and Gosling soon obtained very promising results. Wilkins thus abandoned all previous studies and concentrated on optimising DNA fibre diffraction patterns. The diffraction pattern produced when a beam of X-rays falls on a crystal in which the molecules are regularly arranged in three dimensions is not a conventional photograph. It shows a set of spots of varying intensity; inferring the structure from the pattern is not a direct process. Each spots corresponds to a diffracted wave from the molecules lying in a particular set of planes in the crystal. The pattern of diffracted waves thus depends on the particular setting of the crystal relative to the incident X-ray beam. The molecular structure of the crystal can be reconstructed mathematically from the amplitudes and phases of all the diffracted waves, obtained by rotating the crystal into different settings. Amplitude means the strength of the wave, which is measurable from the spot intensity, and phase means the positions of the peaks and troughs of the wave relative to some reference point. However, phase is lost in the recording, leading to the so-called phase problem in X-ray crystallography and thus methods had to be developed for determining these lost phases indirectly. For small molecules, analytical methods were developed, but only in 1953 did Perutz solve the problem for large molecules like proteins by a new method.
Very soon Wilkins and Gosling obtained photographs “containing dozens of well-defined spots on a clear background which was a very good result [because] it showed us clearly for the first time that DNA was truly crystalline, and we could be very hopeful that its structure could be derived from X-ray patterns... Signer's excellent, pristine DNA was, so to speak, shouting at us, 'look how regular I am!'.” In his Nobel lecture Wilkins immediately remarked that, on the other hand, they knew that, “genes had to be complicated and therefore DNA had to be complicated.” It should not be forgotten that before 1950 many people received conclusions about DNA being the fabric of genes with some scepticism. There were many fierce antagonists of the idea that DNA played a central role in heredity. Too simple an X-ray diffraction pattern would provide additional evidence that DNA did not have enough complexity to be the genetic material. However, from a decisive set of experiments by the American microbiologists Alfred Hershey and Martha Chase completed in early 1952, it had been definitely established that DNA directs the biosynthesis of enzymes and thus controls the biochemical processes of the cell.
When Wilkins set up to work with DNA, there were already many different indications of simplicity and regularity in its structure. Chemists had definitely shown that DNA was a polymer in which some parts of the molecule were regularly repeated in a polynucleotide chain. And it was also known that DNA included different amounts of the four bases adenine, thymine, guanine and cytosine, but nobody had the slightest idea of what the molecule might look like. In 1947, Chargaff discovered an important regularity: although the sequence of bases along the polynucleotide chains was complex and the base composition of different DNA's varied considerably, the numbers of adenine and thymine groups were always equals, and so were the numbers of guanine and cytosine. In the electron microscope, DNA was seen as a uniform unbranched thread of diameter about 20 angstrom. In 1937, William Astbury at Leeds started pioneer X-ray diffraction studies of DNA fibres and found evidence of considerable regularity. In 1947, he had deduced that nucleotides must be stacked along the fibre axis 'like a pile of pennies' each 3.4 angstrom thick. Thus the conclusion that this chemical substance was invested with a deeply significant biological activity coincided with a considerable accumulation of knowledge concerning its exact nature.
Working closely with Gosling, Wilkins began to obtain detailed X-ray diffraction data of unprecedented quality of what we now know as the A-form of DNA, presenting 100 diffraction spots. His initial studies produced the first clear X-ray images of DNA. They indicated a degree of crystallinity that raised the possibility of a molecular interpretation by X-ray analysis. This was the only type of data that could provide an adequate description of the three-dimensional configuration of the molecule.
As soon as good diffraction patterns were obtained, the mathematician Alex Stokes at King's easily recognised the fingerprints of helical structures, having already encountered them working with clay minerals. He thus worked out the theory of diffraction by a helix. Ideas on helical structures in biological molecules were circulating at that time, especially in Bragg's Laboratory at Cambridge. In 1948, Linus Pauling had predicted the alpha-helix as a secondary structure of proteins. Pauling had deduced this structure from X-ray patterns and from attempts to physically model the structures. Inspired by Pauling's publication on the alpha-helix, Wilkins searched in his experimental data for evidence that DNA was also helical, trying to reconcile the diffraction and biochemical data. From then on, Wilkins and his colleagues continued their research based on the working hypothesis that DNA molecules were helical.
Wilkins presented his early photographs in Naples, Italy, in May 1951, at a conference attended by James Watson. Watson was a younger member of the “phage group,” led in the USA by Max Delbrück, Salvador Luria and Alfred Hershey. This group, formed in 1945, studied bacterial viruses, bacteriophages, using a characteristic mathematics and physics-oriented approach to biology mainly inspired by Delbrück, a German physicist, who had been assistant to Lise Meitner in Berlin, but had moved to biology in the late 1930s, after attaining a fellowship form the Rockefeller Foundation at Caltech. The phage group gave a fundamental contribution to bacterial genetics and to the very origins of molecular biology and the three shared the 1969 Nobel Prize in Physiology or Medicine "for their discoveries concerning the replication mechanism and the genetic structure of viruses."
In October 1950, Watson had moved to Copenhagen to learn nucleic acid chemistry, but he was so inspired by Wilkins' slides that he converted to a structural approach and decided to move to Cambridge to learn X-ray diffraction techniques under Lawrence Bragg. Watson was already convinced that DNA was the genetic material; moreover, Wilkins' photographs showed clear evidence that it had a simple, regularly repeating structure. He moved his fellowship to the Medical Research Council Unit headed by Max Perutz, where the structures of the proteins haemoglobin and myoglobin were being tackled. Watson arrived there in September 1951 and soon met the young physicist Francis Crick, who was interested in interdisciplinary approaches to biology and was working for his PhD on haemoglobin under Perutz. Crick was like-minded concerning the importance of DNA, even if he was more involved in the study of proteins.
In that same year, 1951, another important actor of this story arrived at King's College. While Wilkins and his group were beginning to work on DNA on a broader front, Randall had endorsed the application of Rosalind Franklin, a physical chemist at the time working in Paris, where she had been studying the structure of carbon in various forms using X-ray diffraction methods, a field in which she made very important contributions. Randall's objective was a more systematic X-ray investigation of DNA fibres. Unfortunately he left an ambiguity about the respective positions of Wilkins and Franklin, which led to dissension about the demarcation of the DNA research at King's. The confusion over Franklin's and Wilkins's roles in relation to the DNA effort later developed into considerable tension. Very different working methods and philosophy of research, as well as a series of sharp exchanges, resulted in there being little direct communication. The precious store of the Signer DNA was given to Franklin, who would also supervise Gosling's PhD work on the A-DNA. As a consequence, Wilkins had to acquire new DNA samples from other sources. This division of the research work, fragmentation, and non-cooperative action led on the whole to a lack of coherence and momentum of the King's College project.
During the first year of work, Franklin made important improvements in the preparation of the DNA fibres and assembled a new X-ray tube with Gosling, being able to obtain sharper diffraction patterns. Franklin also made a crucial advance showing that, depending on the water content of the fibre specimen, two forms of the DNA molecule actually existed, which she later labelled A and B defining the conditions for the transition between them. It became clear that all previous workers had been working, without knowing it, mostly with a mixture of the two forms. This explained for the first time the difficulties of earlier workers who had been attempting to interpret such mixtures as a single phase.
By September 1951, Franklin had already obtained X-ray patterns that showed clear evidence of a helical structure. In May 1952, she obtained what became famous as picture B51, a photograph that was to play a crucial role in the final stage of the discovery of the three-dimensional structure of DNA. The characteristic feature of the B-form is the X-shaped pattern of streaks arranged in a set of layer lines, from which it can be directly deduced that DNA in this form is a helix with an axial repeat of 34 angstrom and an axial spacing between nucleotides of 3.4 angstrom. The A form does not show the characteristic X, but there is a detail in the pattern that Franklin recognised to be consistent with a helical structure. Franklin focused on the difficult analysis of the more complicated A form, thinking that, if correctly interpreted, the A pattern would yield more precise information about the DNA molecule. In Franklin's draft notes, prepared for a colloquium on her work she gave in November 1951 at King's College, it is clearly stated that both DNA forms are likely to be a helical bundle of two or three chains. She realised, too, that any correct model must have the phosphate groups on the outside, accessible to water. The group of chains would be linked together by hydrogen bonds between the bases, which would be in the centre of the molecule. She then continued following a strict analytic crystallographic approach on the A form to unveil DNA’s three-dimensional structure.
In the meantime, Watson and Crick often discussed all aspects of DNA with Wilkins - who was a personal friend of Crick's - during Wilkins' visits to Cambridge and their own visits to King's College in London. Watson and Crick heard from Wilkins about his results, also indicating a helical structure in DNA.
After Watson attended to Franklin's enlightening seminar of November 1951, they decided to test their ideas on the possible structure of DNA. Inspired by Pauling's technique they began to build a three-dimensional stick-and-ball model, also basing on what Watson remembered about the structural information from Franklin's talk. After a week, in late November 1951, Wilkins, Franklin and other colleagues were invited to Cambridge to see a three-chain model of the DNA molecule, with the phosphates on the inside and with the bases pointing outwards that Watson and Crick had just built. Franklin immediately expressed complete disagreement, saying that their model did not fit the diffraction data or rules of chemistry. After this failure, Lawrence Bragg, the head of the Cavendish Laboratory, firmly vetoed any further work on DNA.
At King's College in London Wilkins had continued to work on the B-form, working on sepia sperm and getting much clearer patterns than the previous year. But his new DNA samples were not as good as the original ones he had obtained from Signer in 1950, and which Franklin had continued to use. They very clearly offered strong evidence for a helical structure for DNA. Franklin, too, continued to work on her step-by-step detailed analysis of her diffraction data. Since when she had obtained her clear photograph of the B form in may 1952, Franklin had concentrated on the A-form. During the winter 1952-1953 she had some doubts about the helical nature of the A-form, only because of some new experimental results which later turned out to be misleading. From her notebooks it is clear that by March 1953 she was applying helical diffraction theory to the B form and was in favour of two chains per molecule. But since the spring of 1952 she had received permission to transfer her fellowship to John Bernal's laboratory at Birbeck College, also in London, where she moved in March 1953.
In the meantime, Pauling, too, was eager to solve the three-dimensional structure of DNA, as he had already successfully done with other biological molecules. In the summer of 1952, as soon as he learned about Herschey and Chase experiments establishing that DNA controls the biochemical processes of the cell, he immediately switched his attention to this substance, feeling that it should be possible to decipher its structure by building models along similar lines to those in his protein work.
During the last week of January 1953, Pauling's son, Peter, who was at the time working as a graduate student in the Cavendish Laboratory, showed Crick and Watson a draft of his father's paper, written with Robert Corey, in which DNA was described as a triple-stranded helix. The three strands were twisted around each other in ropelike fashion, with the phosphoric acid groups triangularly arranged in the centre and with the various bases pointed outward. The analysis was unfortunately based on rather poor photographs published many years before by Astbury and on equally poor photographs made in their laboratory. Having worked on a similar idea, Crick and Watson immediately had clear what was wrong with a triple stranded helix as proposed by Pauling. This happy circumstance gave them new chance to interpret the structure first. Bragg was persuaded to unleash Crick and Watson from his earlier ban.
In January 1953 Wilkins himself saw Franklin’s Photograph 51 from spring 1952, and in early February, when Watson gave him a copy of the Pauling manuscript, Wilkins showed him such X-ray picture of the B form, with its superior B-form diffraction patterns and its clear helical features.
After their initial failure Crick and Watson were again feverishly working on DNA, encouraged by Franklin's excellent photograph and also remembering her arguments for the backbones being on the outside of the molecule and the bases on the inside. In the second week of February, Max Perutz, a member of the Medical Research Council Committee, and Crick's PhD supervisor, showed him a progress report from King's College that included Franklin's report to that Committee of December 1952. It confirmed much of what they already knew, but the key information Crick derived from the report was related to the fact that the space group symmetry of the A form was C2. Crick immediately drew the conclusion that the molecule should possess a twofold axis of symmetry, thus pointing to two chains running in opposite directions and definitely excluding a three-chain structure. The assignment to A-DNA of space group C2 meant in fact that its structure would comprise two more-or-less identical polynucleotide chains, dyadically paired (that is, anti-parallel) packed like a bundle of cylinders, which were to be thought of as spirals with screw symmetry. Apparently, nobody at King's has enough formal training in crystallography to infer that the DNA molecules must therefore possess perpendicular dyads. Crick immediately understood this fact, because the space group of the crystal of horse haemoglobin on which he had been previously working was C2, identical with that of the A form of DNA. There must indeed be two chains, running in opposite directions. Further details indicated what the rotation angle should be, a critical step in getting the backbone structure right.
A real breakthrough in their work had also occurred in 1952, when Erwin Chargaff visited Cambridge and inspired Crick with a description of his experiments published in 1947. Chargaff had observed that the proportions of the four nucleotides vary between one DNA sample and the next, but that for particular pairs of nucleotides - adenine and thymine, guanine and cytosine - the two nucleotides are always present in equal proportions.
Moreover, in the last week of February, Jerry Donohue, an excellent structural chemist, sharing an office with Watson and Crick, set them on the right track about the correct position of hydrogen atoms, essential for cross-bonding. This allowed Watson to fit in pairs of adenine-tymine and guanine-cytosine as a pair. All this information played a key role in Watson's final insight: the adenine-thymine bond was exactly as long as the cytosine-guanine bond. Chargaff's ratios now automatically arose as a consequence of Watson's base-pairing scheme. The structure of DNA was solved.
When they finished it in early March, Wilkins was the first person outside of Cambridge to see the double helix. According to their three-dimensional model, the double helix of the DNA molecule consists of two antiparallel strands of deoxyribose phosphate (alternating units of sugar and phosphate) on the outside, joined by pairs of bases within the helix. As stated by Chrargaff, adenine is paired with thymine, guanine with cytosine, and the bases to one another by hydrogen bonds. When Franklin saw the model, she had enough elements to understand that it was a reasonable model, because in the meantime she had arrived very near to the solution, as can be inferred from her notebooks and from two papers sent to press before Franklin knew of the Watson-Crick model.
After a whole afternoon of difficult conversation about how much the King’s work had helped Watson and Crick, they agreed that Wilkins and his colleagues would publish their data jointly with Watson and Crick’s announcement in three papers with continuous pagination in Nature. The three papers appeared on 25 April 1953, grouped together under the title “Molecular Structure of Nucleic Acids”. Crick and Watson acknowledged that they had been “stimulated by ... the unpublished results and ideas” of Wilkins and Franklin. Gosling and Franklin added a short note describing the helical evidence in the B photographs, and Wilkins co-authored his paper with Stokes and H. R. Wilson. They gave a preliminary report on the experimental evidence for the polynucleotide chain configuration being helical in the natural state. During the following 10 years Wilkins led a team that performed a range of meticulous experiments to establish the helical model more rigorously.
After Watson and Crick had seen the two King's College papers showing how strongly X-ray evidence supported their structure, they wrote a second article published on May 30 containing the first clear statement on DNA carrying the genetic code: “... it therefore seems likely that the precise sequence of bases is the code which carries the genetic information”.
The Crick-Watson model enabled scientists to explain the process by which DNA replicates itself. The two parts of the DNA molecule separate from each other at the points of hydrogen bonding, somewhat like a zipper unzipping. A new molecule is then synthesised opposite each one on the original strand, with the sequence of bases acting as a code for the formation of new DNA molecules. DNA carries the genetic information of a cell and consists of thousands of genes. Each gene serves as a recipe on how to build a protein molecule. When proteins are needed, the corresponding genes are transcribed into RNA and the transported out of the nucleus. Unveiling the coding of genetic information and understanding the detailed mechanisms of these processes required years of skilful investigations. At the time, the validity and importance of the double helix was quickly recognised by the scientific community, and its discoverers were given numerous awards. Wilkins was elected to the Royal Society in 1959, and he shared the 1960 Albert Lasker Award with Watson and Crick. Two years later, they were awarded the 1962 Nobel Prize in Physiology or Medicine for “discoveries concerning the molecular structure of nucleic acids and its significance for information transfer in living material.”
Unfortunately, by that time Rosalind Franklin had died. After moving to Bernal's laboratory at Birkbeck College, she did very fruitful work on the tobacco mosaic virus and also began work on the polio virus. In the summer of 1956, she became ill with cancer. She died less than two years later at the age of 37. In 2000, King's College London opened the Franklin-Wilkins Building in honour of Franklin's and Wilkins' work at the college.
Bibliography
Kibble T. W. B. and Shallice T. (2006) Maurice Hugh Frederick Wilkins. 15 December 1916 -- 5 October 2004. Biographical Memoirs of Fellows of the Royal Society 52: 455-478
Klug A. (1968) Rosalind Franklin and the Discovery of the Structure of DNA. Nature 219: 808-810, 843-844
Klug A. (2004) The Discovery of the DNA Double Helix. Journal of Molecular Biology 335: 3-26
Selya R. (2008) Wilkins, Maurice Hugh Frederick. Complete Dictionary of Scientific Biography. Vol. 25. Detroit: Charles Scribner's Sons: 298-301. Gale Virtual Reference Library. http://go.galegroup.com/ps/i.do?id=GALE%7CCX2830906208&v=2.1&u=mpi_vb&it=r&p=GVRL&sw=w&asid=7a7ca9c4b48a1f4c4854cc7a746c6278
Wasson T. (ed.) (1987) Wilkins, Maurice H. F. In Nobel Prize Winners, H. W. Wilson Company, New York, pp. 1127-1129
Wilkins. M. (1962) The molecular configuration of nucleic acids, Nobel Lecture, December 11, http://www.nobelprize.org/nobel_prizes/medicine/laureates/1962/wilkins-lecture.pdf