Prof. Dr. F. Sherwood Rowland > Research Profile
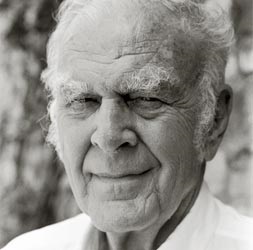
by Luisa Bonolis
F. Sherwood Rowland
Nobel Prize in Chemistry 1995 together with Paul J. Crutzen and Mario J. Molina for "for their work in atmospheric chemistry, particularly concerning the formation and decomposition of ozone".
Going into nuclear chemistry
F. Sherwood Rowland was born in 1927 in the small central Ohio town of Delaware. He was such a brilliant and precocious student that he graduated a few weeks before his sixteenth birthday. During the summers of his early teens, the high school science teacher entrusted to him during his two-week vacations the operation of the local volunteer weather station, where he collected data on daily maximum and minimum temperatures and level of precipitation. It was a precious first experience with systematic experimentation and data collection. After graduation from high school in 1943, Rowland enrolled at Ohio Wesleyan University and in June 1945, as he approached his 18th birthday, he was already eligible for his final year of university. However, with the war in the Pacific still ongoing, he enlisted in a Navy program and began training to become a radar operator. When the Pacific war ended, he served the next year in several Midwestern Naval Separation Centres where he devoted a major amount of time to competitive athletics. This period away from academic life convinced him, at age 19, that there was little reason to seek a quick end to his undergraduate education. He therefore arranged his schedule to take two more years rather than one to graduate, and continued to play basketball on the university team.
In fall 1948, he finally received a B.A. degree with majors in chemistry, mathematics, and physics and then applied to the Department of Chemistry at the University of Chicago, the school where both of his parents had earned their Ph.D. degrees. It was under the football stands at the University of Chicago that Enrico Fermi had built the first experimental nuclear reactor in 1942. After the war the university had managed to recruit many of the leading scientists from the Manhattan Project to the Physics and Chemistry departments. At that time, the Chemistry Department of the University of Chicago had a policy of immediately assigning each new graduate student to a temporary faculty adviser prior to the choice of an individual research topic. Rowland was assigned to Willard F. Libby, who had just found a way to use the radioisotope carbon-14 to determine the age of prehistoric plants, animal remains and archaeologic artifacts - a discovery for which he received the 1960 Nobel Prize for Chemistry.
Rowland experienced the sense of excitement that pervaded the University of Chicago at the time and was especially lucky in having excellent teachers like Harold Urey and Edward Teller for physical chemistry and Libby for radiochemistry. He also attended courses on Nuclear Physics given by Maria Goeppert Mayer and by Fermi. The latter was the unquestioned intellectual leader of the Physics Department and it was a widespread belief, also among chemistry students, that one should go to any lecture given by Fermi on any subject. Urey and Fermi already had been awarded Nobel Prizes, and Libby and Mayer were to receive theirs in the future.
While Rowland was working on his thesis concerning the chemical state of cyclotron-produced radioactive bromine atoms, his longstanding interest in athletics continued unabated in graduate school. He finished his Ph.D. thesis in August of 1952 and went off to Princeton University in September of that year for his new position of Instructor in the Chemistry Department. During the period 1953-55, Rowland spent the summer in the Chemistry Department of the Brookhaven National Laboratory where he made some experiments in tracer chemistry. He put a powdered mixture of glucose and lithium carbonate into the neutron flux of the Brookhaven nuclear reactor that resulted in a one-step synthesis of radioactive tritium-labeled glucose. When a nuclear reaction takes place, large amounts of energy are liberated, much more energy than is usually required to break chemical bonds. When an atom is released from a molecule by such a process, its excess energy may be manifested by large amounts of translational energy. This so-called “hot atom” is moving so fast, that it may break other bonds and induce further chemical reactions when it collides with other atoms or molecules. Indeed, the amount of energy available may be so great compared to ordinary “thermal” atoms and molecules, that reactions that could not occur under thermal conditions will happen, leading to production of unusual species that cannot otherwise be generated, permitting studies of the chemistry of unique species. The new sub-field of tritium “hot atom” chemistry attracted the attention of the Atomic Energy Commission and resulted in support for continuation of Rowland's research for many years to follow.
In 1956 he moved to an Assistant Professorship at the University of Kansas, where a new chemistry building including special facilities for radiochemistry had been completed. His research group was soon joined by a series of excellent graduate and postdoctoral research students, including many from Europe and Japan. This research group was very productive for the next eight years, chiefly investigating the chemical reactions of energetic tritium atoms, but every few years the work was extended to some new, challenging aspect of chemistry - first, radioactive tracer photochemistry, using tritium and carbon-14; then chlorine and fluorine chemistry using the radioactive isotopes 38Cl and 18F.
In 1964, Rowland accepted an offer to start up the chemistry department at a new University of California campus in the then-unbuilt city of Irvine and became its first chairman.
Cross-fertilization between Chemistry and Meteorology
In 1970, Rowland decided to retire from the Chemistry department chairmanship and began looking for new research paths. At the time, the state of the environment had become a significant topic for discussion both by the general public and within his family. For this reason, he participated in an International Atomic Energy Agency (IAEA) meeting on the environmental applications of radioactivity held in Salzburg, Austria. Afterward on the train to Vienna, he shared a compartment with an AEC program officer also coming from the IAEA meeting. Rowland himself recalled the conversation: “He learned in our conversation that I was personally interested in atmospheric science because of my early association and admiration for the work of Bill Libby, and further that my research had then been supported by the A.E.C. for the previous 14 years. I in turn learned that one of his A.E.C. responsibilities was the organisation of a series of Chemistry-Meteorology Workshops, with the intention of encouraging more cross-fertilisation between these two scientific fields.”
During the second of these workshop held in January 1972 in Florida, Rowland heard a presentation about recent measurements by the British scientist, James Lovelock, of the atmospheric concentrations of a trace species, the man-made chlorofluorocarbon CCl3F. Lovelock had earlier invented an extremely sensitive detection system employing electron capture by trace impurities, and attached it to the column of effluent gases from a gas chromatograph, a device which separates a mixture of gases into its individual components. With this apparatus, Lovelock initially established that CCl3F was always detectable in the atmosphere near his home in western Ireland, and then that it was also present in all of the air samples tested on the voyage of the R.V. Shackleton from England to Antarctica.
In the early 1970s, chlorofluorocarbons (CFCs) were a triumph of the chemical industry, already in wide and growing usage around the world. Invisible, nontoxic and nonflammable, and engineered to be chemically inert, these synthetic chemicals were known for their considerable stability and thus widely used as near-ideal coolants in refrigerators and air conditioners, in insulation and as propellants in many aerosol-spray products such as deodorants and hairsprays.
CFC's do not readily undergo chemical reactions. Scientists at that time therefore believed that these molecules caused no harmful effects. Lovelock suggested tracking the CFC's to learn more about the atmosphere. Lovelock's shipboard observations showed the presence of CCl3F in both the northern and southern hemispheres, in quantities roughly comparable to the total amount manufactured up to that date. One of the special advantages cited for this molecule was that it would be an excellent tracer for air mass movements because its chemical inertness would prevent its early removal from the atmosphere. The appearance in the atmosphere of a new, man-made molecule provided a scientific chemical challenge. Rowland wondered: Was enough known about the physicochemical behaviour under atmospheric conditions of molecules such as CCl3F to allow prediction of its fate, once released into the environment?
In early 1973, in submitting his regular yearly proposal to the AEC, which had sponsored his research involving radioactive tracer species since 1956, Rowland also included a predictive study of the atmospheric chemistry of CCl3F: what would eventually happen to the chlorofluorocarbon compounds in the atmosphere?
The atmospheric fate of the chlorofluorocarbon molecules
That same 1973, Mario Molina, who had just completed his Ph. D. work as a laser chemist at the University of California, Berkeley, joined Rowland's research group as a postdoctoral research associate. Offered his choice among several areas for their collaborative research, Molina chose the one furthest from his previous experience and from Rowland's own experience as well, and they began the scientific search for the ultimate atmospheric fate of the chlorofluorocarbon molecules.
Rowland and Molina had clear that, as synthetic molecules, CFCs had been designed to be inert and nonsoluble and were unlikely to be decomposed by common atmospheric processes. But they also realised from laboratory data and chemical insight that the CFCs could not remain inert in the atmosphere forever. After CFCs are released into the atmosphere, they rise slowly and essentially nothing happens in the lower atmosphere, that can be reached only by the relatively low energy, long wavelength portion of the ultraviolet (UV) spectrum. However, it was their considerable stability, that allowed them to waft - one spray at a time - into the upper atmosphere where they would readily absorb highly energetic ultraviolet light (wavelengths below 220 nanometers) that usually causes the decomposition of simple atmospheric molecules. All multi-atom compounds are capable of absorbing UV radiation if the wavelength is short enough, and almost all will decompose after absorbing the radiation.
The search for any removal process that might affect CCl3F began with the reactions which normally affect molecules released to the atmosphere at the surface of the Earth. Several well-established tropospheric sinks - chemical or physical removal processes in the lower atmosphere - exist for most molecules released at ground level: Coloured species such as the green molecular chlorine, Cl2, absorb visible solar radiation, and break apart (photodissociate) into individual atoms as the consequence. Highly polar molecules, such as hydrogen chloride, HCl, dissolve in raindrops to form hydrochloric acid, and are removed when the drops actually fall. Almost all compounds containing carbon-hydrogen bonds, for example CH3Cl, are oxidised in our oxygen-rich atmosphere. However, CCl3F and the other chlorofluorocarbons, such as CCl2F2 and CCl2FCClF2, are transparent to visible solar radiation and all radiation that penetrates to the lower atmosphere. They are basically insoluble in water and do not react with HO, O2, O3, or other oxidising agents in the lower atmosphere. When all of these usual decomposition routes are closed, what happens to such survivor molecules?
CFC molecules in the lower atmosphere are protected against the very energetic UV radiation by O2 and O3 molecules at higher altitudes. In the upper atmosphere, the UV wavelengths below 242 nm can be absorbed by O2, splitting it into two oxygen atoms (O2+UV light-->O+O), thus initiating a sequence of reactions. Each of these O atoms products normally adds to another O2 molecule to form ozone, O3. Collision with some third molecule, M, is needed to stabilise the O3 product (O+O2+M-->O3+M). These ozone molecules in turn can absorb ultraviolet radiation with absorption increasing greatly in strength at shorter wavelengths in the UV and split off an O atom (O3+UV light-->O+O2). Such absorption is especially strong for wavelengths shorter than 290 nanometers. Again, these O atoms usually reform ozone by reacting with O2. Ozone is actually quite chemically reactive and sometimes intercepts the O atoms forming two O2 molecules (O+O3-->O2+O2). This set of four reactions involving O, O2 and O3 was already recognised by Sydney Chapman in 1930. Through these two-step splitting-plus-combination and other processes a balance of ozone is maintained in the atmosphere by which about 3 parts in 107 of the entire atmosphere are present as O3, versus almost 21% as O2.
About 90% of these ozone molecules are present at altitudes between 10 and 50 kilometres, i.e. in the stratosphere, where the mixing ratio of O3 can rise as high as 1 part in 105, while the troposphere contains only 10 % of the atmospheric ozone. The total amount of ozone in the stratosphere is nevertheless rather small; it corresponds to about the number of air molecules contained in a layer 3 mm thick at standard temperature and pressure. The solar UV energy absorbed by O3 is converted into heat, providing a heat source in the 30-50 km altitude range. This heat source creates the stratosphere, the region characterised by an inverted - that is, increasing - temperature profile that rises typically from 210 K at its base at 10-15 km altitude, to roughly 275 K at 50 km altitude.
Because both O2 and O3 can absorb short wavelength UV radiation, no solar radiation with wavelengths < 290 nm penetrates below the stratosphere. The ozone layer in the atmosphere thus performs two important functions: it removes the most energetic fraction of the UV radiation and changes its energy into heat, both creating and maintaining the stratosphere. For nearly a billion years, ozone molecules in the atmosphere have protected life on Earth from the effects of ultraviolet rays. UV-B radiation (280- to 315- nanometer wavelength) from the Sun is partially absorbed in this layer. As a result, the amount of UV-B reaching Earth’s surface is greatly reduced, diminishing risks of skin cancer, cataracts, suppressed immune system and possible damage to terrestrial plant life, single cell organisms, and aquatic ecosystems.
Detailed knowledge of the Earth's atmospheric ozone distribution, seasonally and geographically, was outlined beginning in the 1920s by a series of experimenters, especially G. M. B. Dobson, who began regular measurements with a UV spectrometer. Dobson realised that careful measurements of the relative intensities of solar UV radiation at different wavelengths could be converted into quantitative estimates of the amount of ozone overhead, and his initial work near Oxford established that the amount of ozone varied from day to day and month to month. The modern era of stratospheric ozone studies began in the 1950s with proposals that the detailed ozone source and removal processes might not be in balance. Rocket experiments were well underway, and extensive data were now available for the upper reaches of the atmosphere and for outer space.
A conclusion began to be formed: less ozone, perhaps by a factor of two, was actually present in the atmosphere than would be expected if the Chapman reactions were the only significant contributors to its creation and removal. Because the formation reactions for ozone seemed solidly based, the “missing” factor was more likely that some additional removal process must exist for ozone. In the mid-1960s, first proposals for the missing removal process fell on a free radical catalytic chain initiated by HO in reactions absorbing O and O3 and releasing O2 (HO+O3-->HO2+O2; HO2+O-->HO+O2). However, further measurements of the rates for these reactions showed that this proposal by itself was clearly insufficient to close the gap between the calculated production and destruction rates for ozone. The discussion had, however, expanded the scientific thinking beyond the Chapman reactions, and had brought free radical reactions into the picture.
How did CFCs enter in this stage? The CCl3F molecule was known from laboratory studies to be able to absorb UV radiation at wavelengths < 220 nanometers, but to encounter such solar radiation in the atmosphere the molecule must first drift randomly through the atmosphere to altitudes higher than most of the O2 and O3 molecules - roughly to 25 or 30 km. More than 98% of the atmosphere and 80% of the ozone lies below 30 km altitude. In this rarefied air, the CFC molecules are exposed to very short wavelength UV radiation and decompose with the release of Cl atoms. Because at any given time, only a very small fraction of CFC molecules are found at altitudes of 30 km or higher, the average molecule survives for many decades before it is decomposed by solar UV radiation according to the reactions CCl3F+UV light-->Cl+CCl2F and CCl2F2+UV light-->Cl+CClF2.
In 1974 Rowland and Molina calculated the vertical profile to be expected for CCl3F in the stratosphere. The decomposition rate for this molecule escalates rapidly with increasing altitude in the 20-30 km range so that the estimated average lifetime in the atmosphere was in the range from 40 to 55 years, while for CCl2F2 it was 75 to 150 years. However, once at the top of the atmosphere, the decomposition process is so rapid that a CFC molecule would last at most a few weeks if directly released there.
The answer to their original scientific question was that the eventual fate of the CFC molecules is photodissociation in the mid-stratosphere with the release of atomic chlorine, but on a time scale of many decades. What is the fate of these chlorine atoms at an altitude of 30 km?
The major chemical components of the mid-stratosphere were well known, and their reaction rate constants with atomic Cl had been measured in the laboratory. The newly released single chlorine atom, Cl, resulting from decomposition of CFCs, is chemically very reactive. During research promoted in the early 1970s by the Climate Impact Assessment Program, some researchers had turned their interest to the potential input of reactive chlorine radicals on stratospheric ozone. In the most thorough of these studies, Richard Stolarski and Ralph Cicerone calculated significant ozone depletions if inorganic chlorine were to be present in the stratosphere at a volume mixing ratio of 1 nanomole/mole of air. They were interested in the possible natural release of HCl to the atmosphere from volcanoes, or of man-made chlorine in the exhaust of solid rockets fuels scheduled for propulsion of the space shuttle. Odd oxygen destruction would take place via the catalytic reaction cycle in which first almost all chlorine atoms react with ozone by the reaction Cl+O3-->ClO+O2 forming ClO, another chemically reactive molecule.
The question then moved to the ClO product from the above reaction. What happened to ClO at 30 km? Atomic oxygen is especially abundant in the upper stratosphere between 30 and 50 km altitude. The ClO left by the last step is also chemically reactive and reacts with atomic oxygen to re-release atomic chlorine (ClO+O-->Cl+O2), leaving a second molecule of diatomic oxygen. This reaction of ClO occurs in one or two minutes during the sunlit part of the day. The net result of these catalytic reactions is: O+O3--> 2 O2.
The study by Stolarski and Cicerone, first presented at a conference in Kyoto, Japan, in the fall of 1973 struggled, however, with the problem of a missing chlorine source in the stratosphere, because research had shown that the volcanic source is rather insignificant. But for Rowland and Molina the ClOx chain of reactions now became the key to understand the impact of the release of chlorine atoms from breakdown of CFCs in the stratosphere.
Since the radical Cl atom starts this whole reaction, but is not consumed in the process, it is a catalyst for the ozone destruction reaction. Within three months, they realised that when this catalytic efficiency of about 100,000 ozone molecules removed per chlorine atom is coupled with the yearly release to the atmosphere of about one million tons of CFC's, the original challenging and interesting scientific question had been converted into a potentially grave global environmental problem involving substantial depletion of the stratospheric ozone layer.
One evening, as that realisation was dawning, Rowland’s wife, Joan, asked how his research was going. “It’s going very well,” he said. “But it looks like, I think, the end of the world.” They became fully aware of its seriousness when they compared the industrial amounts of CFCs to the amounts of nitrogen oxides, which control ozone levels. The role of these catalysts of natural origin had been established a few years earlier by Paul Crutzen. In 1970, Crutzen had shown that nitrogen oxides, present at parts per billion levels in the stratosphere, accelerate the rate of reduction of ozone, which occurs by means of catalytic cycles. He had pointed out that emissions of nitrous oxide (N2O), a stable, long-lived gas produced by soil bacteria, from the Earth's surface could affect the amount of nitric oxide (NO) in the stratosphere. Crutzen showed that nitrous oxide lives long enough to reach the stratosphere, where it is converted into NO. In the reactions NO+O3-->NO2+O2 and NO2+O-->NO+O2, nitrogen oxides NO and NO2 react catalytically with ozone, thus accelerating the rate of reduction of the ozone content. Crutzen then noted that increasing use of fertilisers might have led to an increase in nitrous oxide emissions over the natural background, which would in turn result in an increase in the amount of NO in the stratosphere. Thus he recognised that human activity, too, could have an impact on the stratospheric ozone layer. The connection demonstrated by Crutzen between microorganisms in the soil and the thickness of the ozone layer was one of the motives for the later rapid development of research on global biogeochemical cycles. Rowland and Molina decided to exchange information with the atmospheric sciences community. They went to Berkeley to confer with Harold Johnston, who had carried out extensive laboratory studies of the chemistry of nitrogen compounds. In 1971, he pointed out the possible threat to the ozone layer that the planned fleet of supersonic aircraft and supersonic travel might represent, by being capable of releasing nitrogen oxides right in the middle of the ozone layer at altitudes of 20 km. Crutzen's and Johnston's work gave rise to a very intensive debate.
Rowland and Molina publicised their results at a press conference in September 1974, soon after their first paper on the topic was published in the journal Nature. The two scientists showed that when CFC’s rise into the stratosphere, they are bombarded by powerful doses of ultraviolet rays. A single chlorine atom knocked free, they found, can absorb more than 100,000 ozone molecules. More disturbingly, the atoms could linger in the stratosphere for up to a century. This work was the first step in tracing the causal chain linking industrial production of CFCs with global ozone depletion, demonstrating how certain human activities can have environmental impacts far beyond what one might intuit.
Their original calculations about the behaviour of the CFC species in the stratosphere were actually predictions of the vertical distribution because no measurements were then available for any chlorinated species in the stratosphere, and certainly not for the CFCs. During 1975, two different research groups sent evacuated containers equipped with pressure-sensitive valves up on high altitude balloons, and recovered air samples from the stratosphere.
The measured mixing ratios for CCl3F were in excellent agreement with the vertical profiles calculated by Rowland and Molina in the previous year. This fit between theory and experiment demonstrated both that CFCs reach the stratosphere and that they are decomposed there by solar ultraviolet radiation at the altitudes predicted earlier.
In many public appearances, Rowland presented his case that continued use of CFCs would greatly diminish the ozone layer, thereby increasing the intensity of biologically damaging ultraviolet light at Earth's surface. Stratospheric chemistry at the time was based on balloon-borne samplers of trace gases and on one-dimensional models. Nonetheless, the pair measured the ultraviolet cross sections of CFC-11 (CCl3F) and -12 (CCl2F2) in the lab, calculated their photolytic destruction rates in the atmosphere and derived their atmospheric lifetimes as 50–100 years. They reviewed industrial production and emission of CFCs, projected the build-up and release of chlorine atoms in the stratosphere and concluded that ozone depletion was likely and would be long-lived, even with remediation.
Founding atmospheric sciences
A great deal of media and public attention in CFCs ensued, especially between 1974 and 1978, including hearings in state legislatures, city councils, and the U.S. Congress. At the same time, industry representatives at first disputed Rowland’s findings, and many sceptical colleagues in the field avoided him. Opposition from affected industries arose and he was criticised, ridiculed and discounted. One industry group called him an agent of the KGB.
For a decade, Rowland and Molina persevered to prove their hypothesis, publishing numerous scientific papers and sometimes speaking to hostile audiences at scientific conferences. Cicerone, whose own work had established the possibility of a chlorine chain reaction, said: “He [Rowland] mentioned to me that he had not been invited to any chemistry department to give a lecture” from about 1975 to 1985. “The territory they stepped into and defined was so new that most scientists felt they didn’t know what was going on... They didn’t feel prepared - or they felt the linkage with an ongoing human activity was too big a step.”
But their findings, achieved in laboratory experiments, were supported 11 years later when British Antarctic Survey scientists Joseph Farman, Brian Gardiner, and Jonathan Shanklin detected recurring massive springtime losses of ozone over their survey station at Halley Bay, Antarctica. The station had been established in preparation for the International Geophysical Year of 1957-1958 and was equipped with a standard Dobson UV spectrometer for the measurement of total ozone. Since the late 1970s, observations showed that the average October ozone concentrations over Halley Bay had begun to decrease (spring in Antarctica corresponds to fall in the northern hemisphere). In 1984, values had dropped well below the values of the 1960s. It became clear that the loss of ozone began soon after the end of the polar winter darkness and proceeded very rapidly for the next several weeks into mid-October.
This totally unexpected phenomenon was reported in Nature in May 1985, together with the hypothesis that the ozone decrease was correlated with the increasing CFC concentrations in the atmosphere.
Further observations of substantially less ozone over Halley Bay in October in the 1980s were quickly shown to be characteristic of the entire South Polar Region by the total ozone mapping spectrometer instrument on the Nimbus-7 satellite. Measurements taken on other satellites over the entire sunlit Southern Hemisphere showed that the lowest October ozone values fell rapidly during the years. These data provided the scientific basis for the conclusion that the ozone losses over Antarctica develop during the special atmospheric conditions existing in the long cold months of polar darkness. High wind speeds cause a fast-rotating vortex of cold air, leading to extremely low temperatures. Unusual and heterogeneous chemical reactions occur on the surface of the frozen stratospheric cloud particles, driven by the heightened chlorine. About half the total column ozone is lost during austral spring. The spatial scale of Antarctic ozone loss is immense, covering nearly the entire continent and thus this phenomenon has been popularly christened the ozone ‘hole’.
Moreover, during the many decades of random diffusion through the lower atmosphere, these CFC molecules are able to intercept outgoing terrestrial infrared radiation and thereby contribute significantly to the Earth's warming by the greenhouse effect. Svante Arrhenius long ago recognised that the continued combustion of fossil fuels (coal, gas, oil) would result in higher concentrations of atmospheric CO2 and suggested that the incremental trapping of infrared radiation would eventually cause global warming. As part of the International Geophysical Year (I.G.Y.) of 1957-1958, CO2 monitoring stations were established and further measurements provided the experimental evidence that CO2 had been increasing in concentrations over the following decades. In 1975, Ramanethan recognised that the rapid growth in the concentration of CFC-12 and CFC-11 provided two important additional greenhouse gases, because - as with ozone, carbon dioxide and water vapour - they are capable of absorbing the infrared radiation emitted by the earth. The energy of this infrared radiation is not sufficient to destroy the CO2 or CFC molecules when absorbed and is merely converted into heat in the process.
Rowland and Molina’s scientific investigations, together with Paul Crutzen's researches and commitment, started an environmental movement that ended with a success story for global cooperation on a worldwide environmental threat. Regulations for the control of CFC emissions had first been introduced during the late 1970s for the use of CFC-11 and CFC-12 as propellant gases for aerosol sprays, but only in the United States, Canada, Sweden and Norway. Companies continued to produce CFCs for other uses. Moreover, there was no urgency by the international community to ban CFCs outright, and researchers were challenged to prove that the observed ozone depletion was well beyond natural variations. The 1985 discovery of the huge ozone hole above Antarctica answered this challenge and that same year a United Nations meeting in Vienna, Austria, had agreed to a convention concerning protection of the stratospheric ozone layer. After a series of rigorous meetings and negotiations, the Montreal Protocol on Substances that Deplete the Ozone Layer was finally agreed upon on 16 September 1987 at the Headquarters of the International Civil Aviation Organization in Montreal. A landmark international environmental treaty was signed by 70 countries agreeing to phase out the production and usage of CFCs and other ozone-depleting chemicals and to eliminate inventories of them. Today, nearly 200 countries are signatories, including the United States.
Rowland also became very active in evaluating atmospheric ozone data, searching for the predicted decreasing trends. In the late 1970s, he initiated a programme to monitor background concentrations of various gases. With his students he published analyses of air samples from all over the word, showing that atmospheric methane (CH4) concentrations were rising. With his colleague (and former student) Don Blake, he reported measurements in 1982 showing that the greenhouse gas methane was growing in concentration in air samples from all over the world. This finding attracted much attention and demonstrated that human-caused climate change can extend beyond carbon dioxide released from fossil-fuel burning. With Blake, he identified in 1995 that the unusual mix of high ozone and hydrocarbons in Mexico City was due to leaking propane stoves and heaters, rather than traffic.
All this research work was instrumental in starting the field of atmospheric chemistry. The further manufacture of CFCs has been banned by the 1992 revisions of the 1987 Montreal Protocol of the United Nations. Atmospheric measurements have confirmed that the Protocol has been very successful in reducing further emissions of these molecules. Recovery of the stratosphere to the ozone conditions of the 1950s will occur slowly over the years because of the long lifetime of the precursor molecules. Nowadays, models suggest that the concentration of chlorine and other ozone-depleting substances in the stratosphere will not return to pre-1980 levels until the middle decades of this century. On the other hand, it appears that the increasing impact of the greenhouse gas warming will delay or even postpone the recovery of ozone levels in some parts of the globe. The ozone layer over the tropics and mid-southern latitudes might not return to pre-1980s levels of ozone for more than a century, if ever.
Rowland and Molina were jointly awarded the 1995 Nobel Prize for Chemistry with Paul Crutzen “for their work in atmospheric chemistry, particularly concerning the formation and decomposition of ozone.” In announcing the Prize, the Royal Swedish Academy of Sciences said that the researchers had “contributed to our salvation from a global environmental problem that could have catastrophic consequences.” Had CFC emissions not been stopped, we now understand that in addition to their destruction of the ozone layer, their impact on climate (through their greenhouse effect) would be comparable to that of carbon dioxide. These discoveries were about more than just stratospheric ozone, they were about the whole environment. The realisation that something done anywhere on the Earth could have effects somewhere else represented the start of the global era of the environment. Having inadvertently opened Pandora's box, mankind was now faced with the necessity of a full understanding in order to keep its atmospheric environment healthy and productive. As Rowland remarked in 2006, “Beyond the ozone problem, the greenhouse effect, global warming and abrupt climate change are now presenting much more forbidding scientific, economic and political challenges.”
Bibliography
Cicerone R. J. et al. (2012) F. Sherwood Rowland (1927-2012). Science 336 (6078): 170
Prather M. J. (2012) F. Sherwood Rowland. Nature 484: 168
Rowland F. S. (1995) Autobiography. Nobelprize.org. Nobel Media.
http://www.nobelprize.org/nobel_prizes/chemistry/laureates/1995/rowland-bio.html
Rowland F. S. (1995) Nobel Lecture in Chemistry.
http://www.nobelprize.org/nobel_prizes/chemistry/laureates/1995/rowland-lecture.pdf
Rowland F. S. (2006) Stratospheric ozone depletion. Philosophical Transactions: Biological Sciences 361(1469) Reviews: 769-790