Prof. Dr. Max Ferdinand Perutz > Research Profile
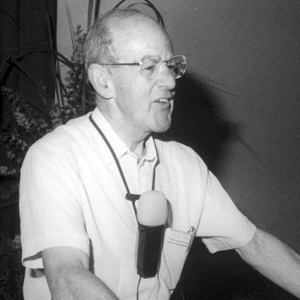
by Luisa Bonolis
Max Ferdinand Perutz
Nobel Prize in Chemistry 1962 together with ohn C. Kendrew
"for their studies of the structures of globular proteins".
The founders of protein crystallography
Max Ferdinand Perutz dedicated a large part of his career to unravelling the molecular structure and function of haemoglobin, the protein of red blood cells, making pioneering use of X-ray crystallography. During his long enterprise, he became a leading figure of the revolution in the life sciences that we now call molecular biology, one of the many fields showing the importance and diversity of applications of diffraction by crystals. The large number of Nobel Prizes awarded for studies involving X-ray, neutron, or electron diffraction is an astonishing demonstration of the incredible impact on the chemical, biochemical, physical, material, and mineralogical sciences of Max von Laue's discovery, going back to 1912.
Perutz was born in Vienna, Austria, in 1914. His parents, both of whom came from wealthy textile manufacturing families, hoped that he would study law and enter the family business, but Perutz developed a strong interest in chemistry while attending secondary school. In 1932, he entered the University of Vienna to study inorganic chemistry, but he found the subject so boring that very soon he shifted to organic chemistry. During his studies in this field he learned about the X-ray crystallography studies then were being conducted at Cambridge University. After completing his undergraduate requirements, in 1936 he went to Cambridge to work under John Bernal, who in 1934 had been the first to obtain clear images of X-ray diffraction patterns of the protein crystal pepsin, after discovering that the crystals had to be kept in their mother liquor. Bernal became the founder of protein crystallography.
The technique of X-ray crystallography originated in 1912, when Max von Laue had expressed the idea that the spacing between crystal lattice planes would be of the right size to cause the diffraction of X-rays, supposing them to be very short electromagnetic waves. Von Laue's hypothesis was brilliantly confirmed by Walter Friedrich and Paul Knipping, by passing a beam of X rays through a crystal onto a photographic plate and producing a pattern of spots. This experiment ended a decade and a half of unsystematic searches for an interference effect in X rays. A few months later, Lawrence Bragg, then a student at Cambridge, visualised this lattice structure as being constructed from a series of sheets of atoms, laid one on top of another, and conceptualised the effect as that of the reflection of X rays by the successive parallel crystal planes, acting like a mirror. Lawrence Bragg also proposed a simple but powerful equation, relating the wavelength of the incident wave, the spacing of the planes in the atomic lattice, and the angle between the incident ray and the scattering planes (the glancing angle) at which constructive interference occurs. This expression became universally known in the community of crystallographers as “Bragg's law.” Bragg’s law (and the notion of reflection) became particularly important in the early development of X-ray crystallography, because it rendered the process of diffraction easier to visualise and simplified calculations.
These early investigations were performed by Lawrence Bragg and his father Henry Bragg, who built an X-ray spectrometer with which they analysed the structure of several salts and small molecules, establishing fundamental mathematical relationships between an X-ray diffraction pattern and the dimensional arrangement of atoms in the crystal that produced the pattern. In their early work, the Braggs made a series of approximations in order to unravel the complex tangle that the reflexions from some crystal represented. What Lawrence Bragg did was to calculate the intensities of all reflections (interference points) in an X-ray diffraction photograph and to compare these values with the observed intensities. From the starting positions of the atoms (based mainly on packing considerations), the atoms were moved until the calculated and observed structure factors were in the best possible agreement. This “trial-and-error” method for structure determination worked because the compounds were simple and the calculation method was very sensitive to the positions of the atoms. The Braggs reduced X-rays analysis of simple materials to a standard procedure by 1914, and both headed a major school of X-ray crystallography between the wars. Their findings created a new science, making it possible to determine molecular structures from the crystal form of a compound.
Von Laue was awarded the 1914 Nobel Prize for Physics for his breakthrough discovery, while the Braggs, father and son, were jointly awarded the 1915 Prize for Physics “ for their services in the analysis of crystal structure by means of X-rays.”
The Braggs led the field of X-ray crystallography for several decades, encouraging a new generation of researchers, including John Bernal and William Astbury, both pioneers of molecular biology. In their initial work the Braggs examined relatively simple crystals, such as sodium chloride, which are composed of only a few types of atoms, while Bernal was interested in the far more complex structure of proteins. He hoped that diffraction studies would eventually enable him to elucidate the function of a given protein.
When Perutz started his X-ray work on crystalline proteins in the Cavendish Laboratory in 1937, Bernal and Dorothy Hodgkin had just demonstrated that protein crystals could be made to yield sharp X-ray diffraction patterns. After learning X-ray diffraction techniques from Bernal and from the physicist Isidor Fankuchen, Perutz began investigating haemoglobin, the globular, oxygen-carrying protein of blood, after a suggestion by the biochemist Felix Haurowitz. Cambridge had a long tradition of haemoglobin research since the beginning of the century. Thanks to this wealth of expertise on the biochemistry and physiology of haemoglobin, and stimulated by Bernal's visionary faith in the power of X-ray diffraction, Perutz pursued his studies in protein crystallography. He used horse haemoglobin crystals, and began his doctoral thesis on its structure. Haemoglobin was a subject that was to occupy him for most of his professional career.
Gradually, it emerged that each unit repeat volume in a crystal of haemoglobin has about 12000 atoms. By 1937, X-ray analysis had solved structures containing no more than about 100 atoms. It took Perutz more than 20 years of hard work to solve the structure of haemoglobin.
In 1938, the Nazi annexation of Austria left Perutz without financial support from his parents. Bragg, who had recently been appointed as Ernest Rutherford's successor to the Cavendish Chair in Cambridge, was quite excited at the prospect of extending the method he himself had first applied to propose a structure for common salt some twenty-five years before, to the fantastically complex structure of proteins. He thus obtained a grant from the Rockefeller Foundation that enabled Perutz to remain at the university as Bragg's research assistant and to receive his doctorate in 1940. This circumstance also enabled Perutz to have his Jewish parents come to Britain and thus escape deportation.
The following year, after Britain entered the war, Perutz, along with hundreds of German-speaking people then living in the UK, was interned first in the Isle of Man, then in Quebec, Canada, as an enemy alien; however, because of his side interest in the crystalline properties of glaciers, he was involved in 1943 in a secret Allied project on the possible use of ice floes as aircraft carriers, a project that was never implemented.
The structure of proteins
After the war Perutz obtained a research fellowship from the Imperial Chemical Industries and resumed his studies of haemoglobin at the Cavendish Laboratory. At that time, Lawrence Bragg organised the research effort at a much larger scale, found support for the project of protein analysis, and assembled a team to tackle these new problems. In his activity as an outstanding scientific organiser, Bragg promoted the establishment of specific laboratories for the research into the molecular structure of biological systems. Perutz was named director of the Unit for Molecular Biology established in 1947 by the Medical Research Council at the Cavendish Laboratory in Cambridge. The Unit attracted more and more researchers who felt that the new field of molecular biology had great promise. As the staff gradually expanded, it included Francis Crick, a brilliant young physicist who became Perutz’s PhD student in 1948, and the geneticist James Watson in 1951. They proposed in 1953 a double helix structure for the polynucleotide chain configuration of DNA, an achievement for which they shared the Nobel Prize in Physiology or Medicine with Maurice Wilkins in 1962.
Initially, Perutz’s only colleague was John Kendrew, a former doctoral student who had been convinced by Bernal of the promises of protein crystallography. He was conducting X-ray studies of the muscle protein myoglobin. Myoglobin is a small, bright red protein that acts as a temporary storehouse for the oxygen brought by the haemoglobin in the blood. It is very common in muscle cells, considerably simpler in structure than haemoglobin, being a quarter its size and consisting of a single polypeptide chain.
All protein molecules contain polypeptide chains and some of them contain no other constituents; in others there is an additional group of different kind. For example, the haemoglobin in red blood corpuscles contains four polypeptide chains and four so-called haeme groups: flat assemblages of atoms with an iron atom at the centre. The function of the haeme group is to combine reversibly with a molecule of oxygen, which is then carried by the blood from the lungs to the tissues. However, a polypeptide chain of perhaps hundreds of links can be arranged in space in an almost infinite number of ways. Chemical methods give only the order of the links; equally important is their arrangement in space, the way in which particular side chains form cross-links to bind the whole structure together into a nearly spherical object (as most proteins are known to be). Also of equal importance is the way in which certain key amino acid units, perhaps lying far apart in the sequence, are brought together by the three-dimensional folding (at the so-called active site of the molecule), enabling the protein to perform its special functions.
How is it possible to discover the three-dimensional arrangement of a molecule as complicated as a protein? The key to the problem is that luckily many proteins can be persuaded to crystallise, and often their crystals are as regular and as nearly perfect in shape as the crystals of simpler compounds. Crystallisation actually implies a regular three-dimensional array of identical molecules. The existence of protein crystals means that proteins do have a definite three-dimensional structure to solve. The most powerful techniques for studying the structures of crystals are those of X-ray crystallography; but protein molecules contain at minimum thousands of atoms, and have enormously convoluted and irregular formations that are extremely difficult to elucidate.
As researchers began to use the Braggs’ X-ray crystallography method to identify increasingly complex structures, they soon discovered its limits. In the 1930s, when Bernal, Dorothy Hodgkin and Max Perutz performed the earliest crystallographic studies of proteins at Cambridge’s Cavendish Laboratory, they soon realised that the intricacies of three-dimensional structure of proteins were too complex for analysis by conventional methods. Each type of crystal has its own characteristic arrangement of atoms and so will produce its own specific X-ray pattern, the features of which can be unambiguously, if tediously, predicted by calculation if the structure of the crystal is known. X-ray analysis involves the reverse calculation: Given the X-ray pattern, what is the crystal structure that produced it? Each spot on a diffraction pattern provides information only about the intensity of the X-ray waves that have been deflected off the atoms in the crystal onto the path leading to that spot. Ideally, researchers also needed to know which particular point in the undulating cycle of movement from one wave peak to the next - known as the phase of the wave - these X-ray waves are at when they hit the film and form each spot in the diffraction pattern. In the case of simple molecules, containing only tens of atoms, the diffraction pattern and wave intensity information was sufficient to identify a structure, with the help of a formula devised by the French mathematician Joseph Fourier in the 19th century, which breaks down complex waveforms into their constituent waves. In analysing complex crystals, the calculation is carried out by applying Fourier synthesis to the repeating three-dimensional configuration. Although it was a painstaking task, researchers in the early years of crystallography could almost always rely on this Fourier synthesis formula, together with a healthy dose of trial-and-error and informed intuition, to hit upon the right structure of these compounds.
However, larger molecules with thousands of atoms, like haemoglobin, posed a much greater problem. The number of reflections and interactions that occur within crystals of this size is so complex that to translate X-ray patterns into molecular structures requires more than just knowledge of the intensity of the waves, which could be directly measured on the diffraction pictures (up to the mid-1950s this was done by eye). To have any chance of deducing the structure, researchers needed to find a way of knowing what phase the X-ray waves are at when they form each spot in the diffraction pattern. This information could be calculated by knowing the phase angle, the distance between a point on a wave and a specified reference point. However, this vital piece of information couldn't be directly obtained from the Fourier synthesis, or indeed any other method and remained missing in the process of making protein structure determinations possible.
In his work on haemoglobin Perutz, too, faced fundamental problems in the interpretation of his X-ray pictures, because they contained only the intensities and not the phases of the diffracted rays. Without knowledge of the phases it was impossible to solve the structure. Researchers called the case of this missing information “the phase problem”, and at the time solving this was the ultimate goal for anyone interested in trying to determine protein structures from X-ray crystallography.
Without this missing phase information, researchers turned to another method that tried to get around the problem. Lindo Patterson devised a variation of Fourier synthesis in the 1930s, developing a method that partially avoided the phase problem by creating a contour map that could be used to define the distances between atoms in a crystal, knowing only the position and intensity data in an X-ray photograph. The upside of these Patterson maps was that you didn't need to know the phases of the reflections, but the downside was that creating a Patterson map was a hugely time-consuming task even for simple compounds, involving hundreds of calculations for each spot in a diffraction pattern. In addition, Patterson maps left ample space for interpretation regarding the actual position of the atoms in space, so that they were not necessarily guaranteed to be successful with more complex compounds. Many workers felt that it was not satisfactory as a direct means of solving protein structures.
Notwithstanding the great development of X-ray crystallography techniques, for many years it was thus not possible even in principle to imagine how the structures of crystals so complex could be discovered. Their X-ray patterns contained many thousands of reflections, paralleling the complexity of the molecules themselves. For their interpretation, there was no hope of proceeding by “trial and error” methods, on which X-ray crystallography techniques still largely depended; the first model could never be good enough to provide a useful starting point. Thus, although protein crystallographers discovered many interesting facts about protein crystals, they did not succeed in extracting much information bearing directly on the molecular structure.
Solving the phase problem
Images of complex molecules proved impossible to interpret until 1953, when Perutz recalled a strategy that his mentor Bernal had proposed more than a decade earlier: the incorporation of heavy atoms - preferably a metal - into haemoglobin crystals altered the diffraction patterns produced by X rays, providing a frame of reference for obtaining the phase relationships of the diffracted waves. Taking X-ray diffraction pictures of crystals identical in shape and molecular structure, but differing in that in one case a heavy atom is present, while in another case it is absent (or replaced by a further heavy atom), the positions of the diffracted beams do not alter, and only the intensities vary. By comparing the original and the modified patterns, it was now possible to determine the location of specific atoms and hence gain important information about the structure of the crystal. The method had been used successfully to solve small organic structures. However, nobody thought that it would be possible to apply it to such huge molecules as proteins, because the X-ray scattering contribution from a metal atom was thought to be too weak to observe, and moreover, inserting sufficiently heavy atoms would distort the protein structure. Perutz did not give up and did some preliminary work that gave him confidence that the method would work in a protein. He found a way to attach mercury atoms to sulphydryl groups on haemoglobin without any change in the oxygen uptake. This indicated that the structure of the molecules most likely had not been altered. He succeeded in crystallising this haemoglobin including heavy mercury atoms and thus his observation for the first time made it possible, in principle at least, to solve the complex X-ray pattern of a protein crystal and to produce a model of the structure of the molecule. The phase problem could be solved. By 1956, he had created half a dozen types of haemoglobin molecules, and collected X-ray diffraction data from six isomorphous derivatives tagged with a heavy atom attached at a different location. This was a turning point in his work and a great breakthrough in protein crystallography. Bragg was so thrilled that he went around telling everyone that Perutz had “discovered a goldmine.” But it took another 5 years before the full three-dimensional structure was obtained at 5.5-angstrom resolution.
Kendrew, working on the smaller myoglobin molecule, was the first to take full advantage of the new method. He too, applied the isomorphous replacement method, even if he found that myoglobin would not retain mercury atoms, so that he was forced to search for other heavy atoms as a substitute, applying a laborious empirical procedure. He was then able to proceed with his collaborators to a study of the three-dimensional structure of the crystal.
Even if the path was now open for a direct structure determination of haemoglobin and myoglobin, there was still an enormous amount of data to be processed. The first tool that allowed the final solving of the structure of haemoglobin and myoglobin was the isomorphous replacement method devised by Perutz, but the second key tool was the electronic computer. Kendrew had to examine 110 crystals and measure the intensities of about 250,000 X-ray reflections. Crystallographers were among the first scientists to use the first generation of valve electronic computers. In 1951, Cambridge University was one of only three or four places in the world with a high-speed stored-program electronic computer. Kendrew took full advantage of the speed of Cambridge’s EDSAC computer, and its more powerful successors, to execute the complex mathematical calculations required to solve the structure of myoglobin. He was actually the first to apply such new tools to the solution of a complex problem in biology. In 1958, Kendrew, working both at the Cavendish and with David Phillips at the Royal Institution, solved the three-dimensional structure of myoglobin, an achievement greeted worldwide as sensational. Considerably simpler in structure than haemoglobin, being a quarter its size and consisting of a single polypeptide chain, myoglobin still posed a formidable challenge, consisting of some 150 amino acid units – making for a total of approximately 2600 atoms - together with a single haeme group.
From the calculated electron density maps, a model of the myoglobin molecule to 6-angstrom resolution was constructed. Calculations completed in the summer of 1957, gave them the density of electrons at a large number of points in the crystal, a high electron density being found where many atoms were concentrated. The model contained a number of dense rod-like features that made up the bulk of the polypeptide chain. The chain was folded in an irregular manner, certainly much more complicated than had been anticipated. In a pocket in the molecule, there was a dense flattened disc, which presumably was the position of the haeme group with its central iron atom. The 6-angstrom resolution was too low to show the molecule’s finer features. Kendrew's group made the following step towards the 2-angstrom resolution.
In the X-ray diffraction patterns of protein crystals the number of spots runs into the tens of thousands. These all have to be measured in several isomorphous compounds, then the results are corrected by various geometric factors and finally used to build up an image. For instance, the calculation of a three-dimensional image of myoglobin at 2-angstrom resolution involved the recording and measuring of about a quarter of a million spots, and in the final calculation about 5x109 figures had to be added or subtracted. Clearly this would have been impossible before the advent of high-speed computers. Both Perutz and Kendrew were very fortunate, because the development of computers always just kept step with the expanding needs of their X-ray analyses.
These key tools eventually led to a three-dimensional map of haemoglobin at a resolution sufficient to distinguish the fold of its four polypeptide chains and the positions of its four haeme. During the next four years, Perutz made thousands of photographic plates and analysed them with the assistance of computers until in September 1959 Perutz and his colleagues, using 40 000 measurements from crystals of haemoglobin and six heavy-atom derivatives, calculated the three-dimensional structure of the molecule.
By 1960, Kendrew and his team were able to obtain a map of the myoglobin molecule at 2-angstrom resolution. To achieve a resolution of 2 Angstrom it had been necessary to determine the phases of nearly 10,000 reflections, and then to compute a Fourier synthesis with the same number of terms. The Fourier synthesis itself (excluding preparatory computations of considerable bulk and complexity) required about 12 hours of continuous computation on a very fast machine, EDSAC II, at the nearby Mathematics Laboratory, probably the fastest computer in the world then. It showed the haeme group in detail, the polypeptide chain and many side chains, including the famous histidine residues that coordinate the haeme. Looking at the map from the appropriate angles, they were able to see this group as a flat object with a region of high density at the iron atom in the centre. There were clear indications that the polypeptide chain indeed assumed a helical shape. Detailed measurement of the spiral density showed that it followed precisely the dimensions of the alpha-helix deduced by Pauling and Corey 10 years earlier, whose existence in both proteins and polypeptides had been immediately confirmed by Perutz himself. In fact, it turned out that about three-quarters of the polypeptide chain in the molecule took the form of straight lengths of alpha-helix.
The publication of Kendrew's findings on the myoglobin molecule at 2-angstrom resolution, along with the 6-angstrom structure of haemoglobin by Perutz and his collaborators in Nature in 1960, truly heralded a new era in biology. The first solution of the three-dimensional molecular structure of these proteins showed that both molecules were built from Linus Pauling's alpha helices, but folded and packed together in a complicated manner that never could have been deciphered by any other technique.
For being the first to successfully identify the structures of complex proteins John Kendrew and Max Perutz were honoured with alacrity by the award of the 1962 Nobel Prize in Chemistry. That same year they moved into the new Medical Research Council Laboratory of Molecular Biology in Cambridge, with Perutz as director, and Kendrew as deputy director and head of the division of structural studies. With structure information in hand they could then explain how haemoglobin in the bloodstream binds and releases oxygen on cue, how it passes its cargo on to the related storage protein myoglobin, and how a single amino acid mutation can produce the catastrophe known as sickle-cell anaemia. Perutz and Kendrew also observed that the folding of helices was identical in myoglobin and the two chains of haemoglobin, and this along with the simultaneously evolving new technique of amino acid sequence analysis established for the first time the concept of molecular evolution. Detailed structural data emerged from the detection of many pathological variants of haemoglobin collected worldwide from a large range of living creatures and numerous humans and the exact symptoms of each mutant gave new information about the function of different features of the haemoglobin molecule. It was the first time that the causes of human disease could be seen at the atomic level. A deep understanding of several inherited diseases enabled Perutz to open up the new field that he himself named 'molecular pathology', adding to our knowledge of molecular evolution.
Solving the structure of haemoglobin was only the beginning for Perutz. The challenge continued. It took him another decade to solve his original target: the molecular basis of haemoglobin activity, which allows the maximum amount of oxygen to be loaded in the lungs, and at the same time enough oxygen to be unloaded in the tissues, where the oxygen concentration is low. He understood that in order to unravel the oxygen-binding function of haemoglobin, he needed an atomic model of both the oxygenated and deoxygenated forms of haemoglobin. An immense task involving measuring several hundred thousand reflections that Perutz and his collaborators completed in 1970, well thirty-years after Perutz had taken the first X-ray picture of the molecule.
Joining forces with the Cambridge protein biochemist Sanger, Perutz applied to the Molecular Research Council for a new Laboratory of Molecular Biology. The laboratory opened on the outskirts of Cambridge in 1962 and Perutz became its first chair and guided the institution for seventeen years, establishing it as a key centre of the new field of molecular biology.
Bibliography
Chadarevian S. de. (2008) Perutz, Max Ferdinand. Complete Dictionary of Scientific Biography. Vol. 24. Detroit: Charles Scribner's Sons, pp. 79-84. Gale Virtual Reference Library.
Hagan W. J. Jr (1993) Max Perutz. In Laylin K. J. Ed. In Nobel Laureates in Chemistry 1901-1992 (American Chemical Society and the Chemical Heritage Foundation), pp. 435-441
Holmes K. C. (2004) Max Ferdinand Perutz. Biographical Memoirs of Fellows of the Royal Society 47: 227-256
Klug A. (2002) Structural Biology and Biochemistry: Max Perutz (1914-2002) Science, New Series 295 (5564): 2382-2383
Perutz M. F. (1963) X-ray Analysis of haemoglobin. Science 140 (3569): 863-869
Strandberg B., Richard E. Dickerson R. E and Rossmann M. G. (2009) 50 Years of Protein Structure Analysis. Journal of Molecular Biology 392: 2-32
Thomas J. M. (2006) Max Perutz: Chemist, Molecular Biologist, Human Rights Activist. Notes and Records of the Royal Society of London 60 (1): 59-65
Wasson, T. (ed.) (1987) Perutz, Max In Nobel Prize Winners, H. W. Wilson Company, New York, pp. 815-817