Prof. Dr. Emilio Gino Segrè > Research Profile
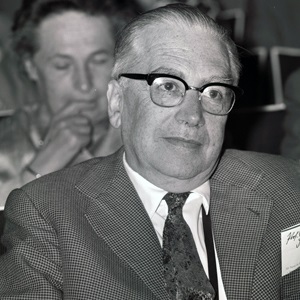
by Luisa Bonolis
Emilio Gino Segrè
Nobel Prize in Physics 1959
together with Owen Chamberlain "for their discovery of the antiproton".
Becoming Fermi's First Student in Rome
Emilio Gino Segrè was born in Tivoli, a small city near Rome where he attended the primary school. After completing his secondary education, in 1922 he enrolled at the University of Rome to study engineering. He was already fascinated by physics, and during the summer 1927 he met Franco Rasetti and Enrico Fermi, who had just settled in Rome coming from Florence. Fermi, in particular, had inaugurated the first ever chair of theoretical physics, created for him by Orso Mario Corbino, the director of the Physics Institute of via Panisperna, who was convinced that Fermi was the person who would give new life to Italian physics. Since some time Segrè was annoyed with engineering, and in fall 1927 he decided to switch, thus becoming Fermi's first student in Rome. The next year he was the first who got his degree under Fermi's sponsorship and in 1930 he was promoted to assistant professor and received a Rockefeller fellowship grant that enabled him to study with Otto Stern in Hamburg and Pieter Zeeman in Amsterdam. Segrè's early research involved atomic spectroscopy - a field in which he had been trained by Rasetti, who was a very skilled experimenter - and later molecular beams and X rays. He made important findings on the spectroscopy of forbidden lines and on the Zeeman and Stark effects, the splitting of lines into several components in the presence, respectively, of a magnetic or an electric field.
The Discovery of Artificial Radioactivity Produced by Neutron Bombardment
At the end of the 1920s/beginning of the 1930s, the Roman group around Fermi, who had in the meantime enlarged with new students such as Ettore Majorana and Edoardo Amaldi, was abandoning atomic spectroscopy, by that time an exhausted research field. They became more and more interested in the intriguing developments, taking place in the realm of nuclear physics, officially inaugurated with James Chadwick's announcement of the existence of the neutron at the beginning of 1932. The subsequent discovery of artificial radioactivity by Irène Curie and Frédéric Joliot in January 1934 immediately aroused Fermi's interest. The French couple had induced artificial radioactivity in light elements bombarding them with alpha particles.
But Fermi thought that neutrons would be much more suitable projectiles, being able to penetrate nuclei without being repelled by the nuclear charge. He immediately succeeded using neutrons provided by small amounts of the gas radon (produced by radium) mixed with beryllium powder.
With an extremely simple equipment, Fermi and his group began with the lightest elements and moved up in the periodic table, coming at last to thorium and uranium, then the heaviest known elements. According to the displacement laws of Soddy and Fajans, the alpha decay should lead to an isotope whose place in the periodic table is two positions below that of the bombarded element, while the daughter of a beta emitter takes the place of the subsequent element. In analysing the chemical end products of the neutron bombardment with the help of the chemist Oscar D'Agostino, they thought that neutron capture resulting in the production of beta-emitting radioisotopes, in the case of the last known elements of the periodic table had led to the production of transuranic elements Z=93 and Z=94.
In fall 1934, during a second series of experiments, Fermi and his associates discovered that by passing neutrons through hydrogen-rich materials like paraffin wax or water, they were slowed down and became much more effective in inducing artificial radioactivity. With this work the group suddenly jumped to the frontier of physics research and Segrè became a pioneer in the field of neutron physics and in the chemistry of radioactive elements, which was opening fundamental perspectives in the realm of their application to biology, medicine and many other fields of scientific, social and technological interest.
In the meantime, Irène Curie and her collaborators in Paris and the physicist Lise Meitner with the radiochemist Otto Hahn in Berlin had also taken up these studies and like Fermi had concluded that the elements produced had to be transuranic elements of atomic number 93 or higher, adding to the growing consensus among scientists. However, already in 1934, Ida Noddack, a chemical expert on rare earths and together with her husband co-discoverer of the element rhenium, suggested that, since the effects of neutron bombardment were still poorly understood, it was conceivable that a different reaction might be occurring, namely breakup of the nucleus into “several large fragments, which could be indeed isotopes of known elements, but not neighbours of the irradiated elements.” Her suggestion probably appeared as a speculation, and certainly because her idea went against the conventional wisdom that no particles heavier than alpha particles could be emitted, it was not taken seriously at the time. Moreover, she and her husband insisted on their detection of element 43, whose main properties had been already predicted by Dmitri Mendeleev, that they had called “masurium”, and which nobody was able to reproduce, a circumstance that undermined considerably their scientific credibility.
Filling a Gap in the Table of Elements: the Discovery of Technetium
The problem of element 43 was taken up by Emilio Segrè himself, who in 1936 was appointed to the chair of experimental physics at the University of Palermo, in Sicily. That same summer he made his first trip to the United States, where he visited the University of California at Berkeley, in particular Ernest O. Lawrence's cyclotron, the dream of Italian physicists at that time. In visiting the Radiation Laboratory he noticed that there was a lot of radioactive metal scrap lying around. Nobody knew what it contained. He asked for some samples to take with him to Palermo. He soon discovered it was a true mine of radioactive substances, especially phosphorus-32 and thought immediately that this might be useful for biological experiments. He began a fruitful collaboration with Camillo Artom, a professor of physiology, in tracer studies of metabolism. In February 1937 he received a letter from Lawrence containing more radioactive stuff, in particular a molybdenum foil that had been part of the cyclotron's deflector. The atomic number of molybdenum is Z = 42. The foil had been bombarded with deuterons, a hydrogen isotope of mass 2 consisting of a neutron and a proton. Segrè well knew the existence of (d, p) and (d, n) reactions, in which one or the other of the projectile’s nucleons is captured by the target nucleus. In the (d, n) process, the proton is captured to create a nucleus with Z = 43. Alternatively, the (d, p) process could lead to a radioactive isotope of molybdenum that undergoes beta decay to produce again Z = 43, the elusive “masurium”, allegedly detected by the Noddacks. Segrè enlisted his experienced chemist colleague Carlo Perrier to attempt to prove through comparative chemistry that the molybdenum activity was indeed Z = 43, an element actually not existent in nature because of its instability against nuclear decay. In this work they had discovered the first chemical element created artificially, but they decided not to name it at the time, also to avoid controversy with the Noddacks. Only after the war, when nuclear reactors produced macroscopic amounts of element 43, Segrè had the satisfaction of seeing, not only that they had made no mistakes, but also that they had found the main properties of the new substance and they gave it the name technetium, after the Greek word technetos, meaning “artificial.” We know today that the longest-lived isotopes of technetium have a half-life of 4.2 million years, a time too short to permit survival from primordial material. It was detected spectroscopically in red giant stars in the early 1950s, thus supporting the theory that stars can produce heavier elements. Its short-lived gamma ray emitting nuclear isomer - technetium-99m - is used in nuclear medicine for a wide variety of diagnostic tests.
An Illustrious Emigrée
Segrè made a second visit to Berkeley in the summer 1938, to investigate short-lived radioactivities from cyclotron bombardments. He expected to return to Italy in the autumn. Instead, nine years were to elapse before he revisited his country. From a short but chilling newspaper item he learned of the new charter of anti-Semitism in Italy. At that time the Italian government of Mussolini passed anti-Semitic civil service laws, and Segrè, a Jew and long time opponent of the regime, found himself catapulted into a precarious new situation. He decided to remain in the United States, but it was not easy to find a new position in a market already saturated by colleagues like Hans Bethe, Felix Bloch, Edward Teller and many others, who had left Europe already in 1933, after the enactment of the anti-Semitic laws in Germany. In 1938 Italian physics, too, received a severe blow, because so many scientists were involved, also as opponents to the politics of Mussolini. That same year Fermi was awarded the Nobel Prize in Physics for his work on artificial radioactivity induced by neutrons and from Stockholm moved to the United States with his family to escape the racial laws that affected his wife Laura.
Lawrence helped Segrè, appointing him a paid research associate at the Berkeley Radiation Laboratory. In the meantime the discovery of fission published in January 1939 by Hahn and Strassmann definitely clarified the puzzle of the transuranic elements so that research at the Radiation Laboratory focused on the high end of the periodic table. That same year Lawrence was awarded the Nobel Prize in Physics “for the invention and development of the cyclotron and for results obtained with it, especially with regard to artificial radioactive elements.” When the 60-inch cyclotron began operating in 1940, Segrè, Dale Corson and K. R. MacKenzie bombarded bismuth with alpha particles synthesizing an artificial element with atomic number 85, the last of the halogens, which filled another gap in the periodic table and was named astatine. Later, starting from 1940, Segrè participated with Glenn Seaborg and others to the discovery of plutonium 239, a new fissionable element, which was synthesized in large quantities starting in 1944, eventually becoming the prime source of energy in the nuclear bomb dropped on Nagasaki, Japan, in August 1945.
The outbreak of war between the United States and Italy made Segrè an “enemy alien,” but by that time his competence in nuclear physics and chemistry was too precious for the war effort, and in the spring of 1943 he was invited by J. Robert Oppenheimer, the scientific leader of the Manhattan Project, to join the vast secret effort to develop the bomb. In the Los Alamos secret Laboratory he headed a group including Owen Chamberlain and Clyde Wiegand, to measure the spontaneous fission rates of uranium-235 and plutonium-239.
In May 1945 they received news of Hitler's suicide, of the surrender of Germany and of the end of the war in Europe. One of Segrè's reactions was: “We have been too late.” For him Hitler was the personification of evil and the primary justification for the atomic bomb work. This feeling was shared by many of his colleagues, especially the Europeans. Doubts arose, and were discussed in many private conversations. But the efforts to complete the bomb continued unabated, and on July 14, 1945, the first detonation of a nuclear device took place in the New Mexico desert. In August, the terrible decision was taken by the U.S. Government to drop two nuclear bombs on the Japanese towns of Hiroshima and Nagasaki. Japan surrendered, and this put an end to the war, but, as Oppenheimer well expressed it: “The physicists felt a peculiarly intimate responsibility for suggesting, for supporting, and in the end, in large measure, for achieving the realization of atomic weapons. Nor can we forget that these weapons, as they were in fact used, dramatized so mercilessly the inhumanity and evil of modern war. In some sort of crude sense which no vulgarity, no humor, no overstatement can quite extinguish, the physicists have known sin; and this is a knowledge which they cannot lose.”
From Nuclear Science and War Research to Big-Science with Accelerators
In 1944 Segrè had become an American citizen and had gotten a reputation as one of the leading theoretical and experimental pioneers of modern physics. At the end of the war he returned to Berkeley as a full professor and group leader at the Radiation Laboratory. Two key members of his Los Alamos team, Chamberlain and Wiegand, joined him, Chamberlain on the faculty and Wiegand as a laboratory staff scientist. Segrè found himself free to work according to his tastes, but became rather frustrated in his efforts to continue research in nuclear chemistry and spontaneous fission by the growing influence of Seaborg, who had convinced Lawrence to support a major effort in nuclear chemistry under his direction. Being convinced that it was not possible to collaborate with Seaborg as an equal, Segrè chose to study high-energy interactions of nuclear constituents instead. With his colleagues he began the study of high-energy proton and neutron bombardment of nuclei, particularly hydrogen, at the 184-inch synchrocyclotron, which had started working at the end of 1946. Many people collaborated on the experimental side, including H. F. York, Chamberlain, Clyde Wiegand, and Tom Ypsilantis.
Physics strategy had changed very much after the war. Many experiments were rather obvious and the problem was only to perform them at the highest possible energy and with a clean technique. It had become often possible to make discoveries simply by having more powerful apparatus - not that this counted for little - because to create such tools and to know how to use them was not given to everybody. But of course it was also essential to know which problems are important and promise solution - the more so as the investments in time, money and effort involved in each experiment had grown immensely. Segrè himself had begun with Fermi and Rasetti in Rome with the “string and wax” philosophy, according to which physicists were supposed to build their own very simple experimental devices, blowing glass and working out one's own tools for research. Working conditions were very different from pre-war times; with more money available it was possible to build adequate detectors. And after the war Berkeley was especially suited for high-energy experiments, because its accelerators excelled both in energy and in the quality of their performance.
Hunting for the Antiproton
The problem of existence of antiparticles, which arose in 1928 with Dirac's relativistic theory of the electron, had been confirmed at the beginning of the 1930s with the discovery of the positron in cosmic rays. For this achievement, in 1936 Carl D. Anderson had been awarded one of the Nobel Prizes in Physics, the other one going to Victor Franz Hess, who had demonstrated the existence of an extra-terrestrial radiation long before, in 1912. The extension of Dirac's theory predicting antiprotons was very plausible, but not certain. For many years, experimental physicists had looked for antiprotons in cosmic rays, where large energies occur. But such pair creations are so rare, that no definite conclusions about the existence of antiprotons could be drawn. In 1954 E. W. Cowan, Bruno Rossi and his collaborators at Massachusetts Institute of Technology, and Edoardo Amaldi and collaborators in Rome, had observed, with cloud chambers and photographic emulsions, events which they cautiously assumed to be caused by antiprotons. In the same year the annihilation of an antiproton seemed to have been signalled in a burst of cosmic rays of ten million billion electron volts discovered by Marcel Schein and colleagues of the University of Chicago in a photographic emulsion flown to 100,000 feet altitude.
These observations were not sufficient to establish the existence of such a particle, but the antiproton was in the air and by early 1950s most physicists believed in the existence of both negative protons and antineutrons and expected a decisive demonstration in the near future. To want to search for antiprotons thus required no imagination. With the completion in 1955 of the Bevatron, an accelerator whose energy of 6 GeV was slightly above the threshold for the formation of nucleon-antinucleon pairs from a proton colliding with a nucleon at rest, an opportunity was afforded of proving the existence of the antiproton unequivocally. Several groups in the Radiation Laboratory competed to develop the appropriate experiment to identify the antiproton, which would be concealed in a beam of fifty thousand other particles of negative charge produced by the interaction. The new machine was surrounded by their experimental setups, all of which represented variants on two themes: electromagnetic detection and photographic detection of the rare particles. However, the antiprotons were expected to be relatively few, very short-lived (because they almost immediately contact protons, when both are annihilated), and extremely difficult to detect among the large number of other subatomic particles created by the powerful collisions.
Segrè, Chamberlain and their associates decided to attack the problem in two ways. One was based on the determination of the charge and mass of the particle. The other concentrated on the observation of the phenomena attendant on the annihilation of a stopped antiproton. The stopped antiproton and a proton of the target should mutually annihilate each other, and the rest mass of the two particles should transform itself in one of many possible ways into other particles such as pions. These would leave tracks in a photographic emulsion and the annihilation would thus become evident.
In principle, the experiment to create and detect antiprotons is very simple. A crucial feature is the transportation of a beam of antiprotons to a detector. One subjects a target to bombardment by 6 billion-volt protons and then looks for negatively charged particles with the mass of a proton, emanating from the target. The analysis of the particles coming from the target is carried out by means of a magnetic field, which deviates the negatively and positively charged particles in opposite directions to one another. One now chooses a magnetic field of such strength that it causes all the antiprotons with a definite momentum to move along a definite circular path into a detector placed somewhere along its path. At the same time another detector is placed at a different point along the path of the particle to obtain its velocity. Both the momentum of the negatively charged particle, as given by the radius of its path in the magnetic field and the strength of this field, and the speed of the particle, as given by the time it takes this particle to cover the known distance between the two detectors, can be measured. From the measured momentum and the speed, one can then calculate the mass of the particle using the well-known relativistic formula that relates the mass to the speed and momentum of the particle.
Although the theory of the experiment is simple, it is quite complicated in practice. The alignment of the detectors must be very precise, furthermore other negatively charged particles, such as negative pions and electrons, having the same momentum as the antiprotons could move along the path of the antiproton. Two of these might trigger the two detectors, giving the impression of a single antiproton. Fortunately, mesons must move about ten times faster than antiprotons to have the same momentum. They then are moving fast enough to emit a characteristic Cerenkov radiation, which is not emitted by much more slowly moving antiprotons. A Cerenkov counter can be thus placed in the path of the deflected particles to differentiate between antiprotons and the less massive particles.
With their elaborate arrangement a clear signal was seen of negatively charged particles with the mass of a proton. After accumulating conclusive evidence, Chamberlain, Segrè, Wiegand and Ypsilantis announced their verification of the existence of antiprotons in October 1955 with a Letter to Physical Review. It was the triumph of accelerators over cosmic rays, which had dominated the field of particle physics since the 1930s.
The experiment also established that antiprotons are not produced alone but in proton-antiproton pairs, just as positrons are produced in electron-positron pairs. In collaboration with Edoardo Amaldi's group in Rome, a program for the search and study of antiprotons in emulsions was in fact initiated concurrently with the counter experiments. Its aim was to provide the proof for the annihilation process. This was actually accomplished with the observation of “stars” produced with a visible energy release greater than twice the rest energy of the proton-antiproton pair. Their identity as examples of antimatter was in fact to be confirmed by the observation of their annihilations in nuclear emulsions. Once the proof was provided, the emphasis was shifted to the study of the annihilation process, and the production of secondary particles like pions and K-mesons. These decay at different stages, so that measuring the total energy of all these decay products and showing that it equals twice the mass of the proton times the square of the speed of light becomes rather complicated. This was done, and the evidence of the antinucleon could now be considered conclusive.
The circumstances surrounding the discovery of the antiproton were not without controversy. After thanking E. J. Lofgren, who served as chief of operations for the Bevatron, and other members of the laboratory, in their Letter announcing the discovery, the group acknowledged “useful suggestions” made by Oreste Piccioni in connection with the design of the experiment. Piccioni, who got his degree in Rome under Enrico Fermi in 1938, during the war completed in Rome a remarkable experiment with Marcello Conversi and Ettore Pancini, which represented the first real demonstration that the so-called mesotron of cosmic rays was not the mediator of nuclear forces predicted by Yukawa, because of its too weak interaction with nuclei. Immediately after the war Piccioni was invited by Bruno Rossi at MIT, and in 1948 moved to Brookhaven National Laboratory where he was still working at the time. In 1972, about 15 years after the discovery of antiproton, Oreste Piccioni alleged that in December 1954, during several meetings he described his ideas about detecting the antiproton in much detail. In particular, Piccioni had suggested that, instead of looking for the postulated antiproton by observing its annihilation process - the conventional approach - it sufficed to show that a particle with negative electric charge and a mass equal to the proton's was produced and should be detected by measuring its time of flight. He also discussed a design of the quadrupole lenses starting from the model he had already computed and was in use before 1954 at the Brookhaven Cosmotron. For some time Piccioni believed that they had agreed to do the experiment jointly, but he claimed that later Segrè and Chamberlain had completely excluded him, never giving him credit for his idea. He further claimed that they prevented him from doing anything about it for 18 years by “threatening” and “cautioning” him that if he did, they would deny him access to the Lawrence laboratory.
Actually, immediately after the discovery of the antiproton, Piccioni collaborated in an experiment for the search of the antineutron. It was in fact suggested that the antineutron should be found by investigating the charge-exchange reaction in which a proton and an antiproton give a neutron and an antineutron according to the reaction proton + antiproton → neutron + anti-neutron. In a very ingenious and elegant counter experiment carried out at the Bevatron, Bruce Cork, Glen R. Lambertson, Oreste Piccioni and W. A. Wenzel demonstrated the existence of the antineutron by stepping up the Bevatron's anti-proton production. The product antineutron is recognizable by its annihilation properties, as it forms an annihilation star extremely similar to an antiproton star.
In 1955 the discovery of the antiproton reopened for Segrè the possibility of being awarded the Nobel Prize. In 1951, he had in fact been deeply disappointed not to share in the Nobel Prize in Chemistry, awarded to Edwin McMillan and Glenn Seaborg, “for their discoveries in the chemistry of the transuranium elements.”
In October 1959, when the date for the announcement of the Nobel prizes was approaching, some information had leaked to the press about a week before the final vote, and some Swedish journalists called Segrè saying he was high in the balloting of the awarding committee. On October 26, Segrè heard the announcement on the radio: the Nobel Prize in Physics had been awarded to Segrè and Chamberlain “for their discovery of the antiproton.” However, the discovery paper has four authors (Chamberlain, Segrè, Wiegand, and Ypsilantis). There are those who feel that Wiegand had deserved as the others to share the prize. Why he was not included is not publicly known.
In any case, quite a number of physicists were actually looking for antiprotons; so it is clear that if their experiment had failed, someone else would soon have found them. If the development and scanning of emulsions had not been so time-consuming, the discovery might have come from emulsion work. Many external circumstances, many causes, and many scientists concurred to the final unequivocal demonstration of the existence of the antiproton - and the antineutron. As John L. Heilbron emphasized in the last sentence of his detailed analysis: “Who discovered the antiproton, when, and why? It is not easy to say.”
Bibliography
Chamberlain, O. (1989). The discovery of the antiproton. In L. M. Brown et al. (eds.). Pion to Quarks: Particle Physics in the 1950s. Cambridge University Press, pp. 273-284
Jackson, D. J. (2002). Emilio Gino Segrè (1905-1989). National Academy of Sciences. Biographical Memoirs 81: 1-25
Heilbron, J. L. (1989). The detection of the antiproton. In M. De Maria et al. (eds.). The Restructuring of Physical Science in Europe and the United States, 1945-1960. World Scientific, Teaneck, NJ, pp. 161-217
Piccioni, O. (1989). On the antiproton discovery. In L. M. Brown et al. (eds.). Pion to Quarks: Particle Physics in the 1950s. Cambridge University Press, pp. 285-295
Segrè, E. (1993). A Mind Always in Motion: The autobiography of Emilio Segrè. University of California Press, Berkeley and Los Angeles
Seidel, R. (2008). Segrè, Emilio Gino. Complete Dictionary of Scientific Biography. Vol. 24. Charles Scribner’s Sons, Detroit, pp. 407-411,
http://go.galegroup.com/ps/i.do?id=GALE%7CCX2830906083&v=2.1&u=mpi_vb&it=r&p=GVRL&sw=w
Wasson T. (ed) (1987). Segrè, Emilio. In Nobel Prize Winners, H. W. Wilson Company, New York, pp. 950-952