Prof. Dr. Edwin Mattison McMillan > Research Profile
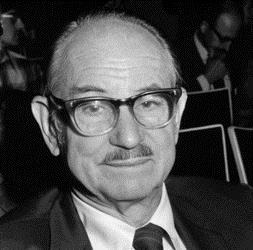
by Luisa Bonolis
Edwin M. McMillan
Nobel Prize in Chemistry 1951 together with Glenn T. Seaborg
"for their discoveries in the chemistry of the transuranium elements".
Education and early work at Lawrence Radiation Laboratory
Given the large scope and fundamental nature of his scientific activity, Edwin M. McMillan can be characterised as a “natural scientist,” keeping up with evolving knowledge in a surprisingly large number of fields. According to Glenn Seaborg, who shared with him the 1951 Nobel Prize in Chemistry, McMillan was in fact one of the most versatile scientists he had ever known, with contributions spanning the fields of physics, chemistry and engineering, who disliked specialisation and the division of physics into theory and experiment, being actually both an imaginative experimentalist and an excellent theorist.
McMillan was born in 1907 in Redondo Beach, California, and was brought up in Pasadena, in the proximity of the California Institute of Technology, where during high school he spent a lot of time around laboratories and attending public lectures and seminars. By the time he entered the California Institute of Technology in 1924, he had developed impressive qualities for scientific research. He took a large number of courses in chemistry, which proved to be instrumental in his later work and was greatly influenced by Linus Pauling, future Nobel laureate in Chemistry for his research into the nature of the chemical bond. McMillan majored in physics and obtained his Bachelor of Science degree and Master of Science degree, later completing his Ph.D. degree in physics at Princeton University in 1932, having received a thorough education in experimental nuclear physics. After winning a National Research Council fellowship for postdoctoral study, he accepted an invitation at Berkeley, where Ernest Lawrence was engaged in exploring the experimental potential of the first cyclotron. After early research on measurement of nuclear magnetic moments and on the hyperfine structure of the solar spectrum, McMillan became progressively more involved with the work on the cyclotron, and his experimental skill was greatly appreciated by Lawrence and his collaborators.
At that time the field of nuclear physics was being transformed by a succession of startling discoveries. In 1934, when McMillan became a research associate in physics at Lawrence's Radiation Laboratory, Frédéric and Irène Joliot-Curie in Paris made the exciting observation that an ordinary stable element could be made radioactive by irradiating it with alpha particles of natural origin. Another extremely important development had taken place in 1932, when James Chadwick, working at the Cavendish Laboratory led by Ernest Rutherford, had finally demonstrated the existence of the neutron, a neutral constituent of nuclei, which Rutherford had hypothesised since 1920, as “almost necessary to explain the building up to the nuclei of heavy elements.” Immediately after Joliot and Curie's discovery, Enrico Fermi in Rome decided to see if neutrons, too, could induce artificial radioactivity. Being uncharged, Fermi thought they would be able to penetrate even into the nuclei of highly packed. He actually obtained positive results in several elements, which became radioactive and decayed with the expulsion of alpha particles, protons, or gamma radiation. According to the displacement laws of Soddy and Fajans, the alpha decay led to an isotope whose place in the periodic table is two positions below that of the parent, that is of the bombarded element, while the daughter of a beta emitter takes the place of the subsequent element.
Since spring 1934, proceeding systematically according to increasing atomic number, Fermi and his collaborators at length also bombarded with neutrons the last known elements in the periodic table, thorium and uranium. As with the other elements, neutron capture resulted in the production of beta-emitting radioisotopes, which in this case could not be easily interpreted. The simplest way was to interpret their results as due to a transuranic element Z=93 generated in the beta decay of a radioactive nuclide produced by radiative capture in uranium (Z=92). The chemistry of the transuranium elements was, of course, not known; it was rather generally thought that they would be like rhenium, osmium, etc., the elements immediately above them in the same vertical column of the periodic table. Therefore, when their chemical tests showed that the induced activities were not due to elements of atomic numbers 86-92, these investigators naturally assumed this to be a transuranic element with chemical properties similar to those of the rare metal rhenium. Soon after, they studied another beta-emitter, suggested to be an isotope of element 94, which they assumed to be homologous to osmium, a transition metal in the platinum family. Fermi's interpretation was for the moment confirmed by Hahn, Meitner and Strassmann working in Berlin, and by Irène Curie and Paul Savitch working in Paris. For several years, the Berlin team interpreted the results on the transuranium assumption; but gradually difficulties piled up to the extent that this interpretation no longer seemed possible.
The possibility of inducing artificial radioactivity had immediately stimulated research toward preparing radioactive forms of many elements. At that time the technology of accelerators was entering a stage of rapid development. Alpha particles from natural sources can penetrate the nuclei of only the lightest elements, while Lawrence's cyclotron, being able to accelerate charged particles to energies far beyond those of naturally occurring sources, made it possible to bombard and transmute the heavier elements for the first time and thus, with time, allowed for the systematic production of radioisotopes used for therapeutic application on human patients. Especially John Lawrence, the physician brother of Ernest Lawrence, soon demonstrated the isotope-making cyclotron's worth in disease research. In 1935, with Lawrence and R. L. Thornton, McMillan studied the radioactivity produced when a variety of targets are exposed to the deuteron beam produced by the Berkeley cyclotron. During that period he did several experiments in what today has become nuclear chemistry and distinguished himself as an innovative and careful experimenter, with an exceptional command of the theoretical background of nuclear physics. He also took a keen interest in the operation and improvement of the cyclotron and was responsible for several substantial improvements in ion sources, magnetic-field shaping, beam extraction, and power and control systems. He developed a deep understanding of the problems related to the energy attainable by cyclotrons and the acceleration of the beam.
Transuranium Elements
Towards the end of the 1930s, the situation in Europe was becoming more and more ominous. As an Austrian citizen, Lise Meitner in Berlin had been protected from the Nazi Civil Service Laws. With the Anschluss of 12 March 1938, those laws suddenly applied to her and she could expect to be dismissed from her post and to suffer other penalties. She was refused an exit visa and a plan was then immediately prepared to get her out of Germany secretly. After she left, Hahn and Strassmann continued the chemical investigation of the radioactivity produced by the bombardment of heavy elements. In 1938, the nuclear scientific community was generally convinced that transuranium elements had been produced in bombarding heavy elements with neutrons. They were treated as an established fact in textbooks, articles, public lectures. In fall 1938, Enrico Fermi was awarded the Nobel Prize in Physics for “for his demonstrations of the existence of new radioactive elements produced by neutron irradiation, and for his related discovery of nuclear reactions brought about by slow neutrons.” The presentation speech, as well as Fermi's Nobel lecture, still mentioned the most probable existence of two transuranium elements, 93 and 94, which Fermi and his group had named Ausonium and Hesperium.
The riddle was finally solved in late fall 1938, when Hahn and Strassmann's experiments led to a new totally unexpected conclusion: uranium bombarded with neutrons splits into fragments consisting of lighter elements, lying very far from uranium in the periodic table, rather than transforming into transuranium elements.
The discovery of fission of uranium announced in early 1939 opened a new era in human history. News of the momentous discovery spread rapidly, creating great excitement and a flurry of experimental research activities. By the end of 1939, over a hundred articles relating to nuclear fission had been published all over the world, inaugurating the advent of the nuclear age. Fermi designed and constructed the world's first nuclear reactor in Chicago and in December 1942 produced the first artificial self-sustaining nuclear chain reaction. In 1944, for “his discovery of the fission of heavy atomic nuclei,” Hahn was awarded the Nobel Prize for Chemistry.
At Berkeley McMillan, like many other scientists, began studies to confirm and to elucidate the new process. In the spring of 1939, he undertook an experiment of a very simple kind: measuring the range of energetic fission fragments by their penetration in a stack of thin foils. The fission fragments came from a thin layer of uranium oxide spread on a sheet of paper, and exposed to neutrons from a beryllium target bombarded by 8 MeV deuterons in the 37-inch cyclotron. After exposure to the neutrons, the sheets were separated and examined for radioactivity by means of an ionisation chamber. The results of the range measurement of fragments were routine; but investigation of the residue in the uranium layer bombarded with deuterons showed in addition to the fragment radioactivity associated with the fission process, the presence of one component with a 23-minute half-life and another of roughly two days.
The first one could with reasonable certainty be ascribed to the 23-minute uranium isotope, uranium-239, already identified by Hahn, Meitner and Strassmann in 1936 as a product formed by a simple neutron capture. But McMillan was especially intrigued by the previously unknown 2.3-day beta activity. He immediately suspected it to be a product of the beta-decay of uranium-239 and therefore an isotope of element 93. According to its hypothesised position in the periodic table, it seemed likely to have chemical properties akin to rhenium. McMillan thus involved Emilio Segrè, who, since his work on radioactivity induced by neutrons with Fermi and his group in Rome, was quite familiar with radiochemistry and especially with the chemistry of rhenium, since he had been in 1937 the co-discoverer with Carlo Perrier of another of its homologs, element 43, actually not existent in nature because of its instability against nuclear decay. Only after the war, when nuclear reactors produced macroscopic amounts of element 43, Segrè and Perrier gave it the name technetium (symbol Tc) after the Greek word technetos, meaning “artificial,” in recognition of the fact that this was the first element to be produced by technical or artificial means. During the summer of 1938, Segrè had been invited by Lawrence and after the enactment of racial laws by Mussolini's fascist regime had remained at Berkeley, participating in research aiming at the production of new elements and isotopes by artificial means. In examining McMillan's 2.3-day activity, Segrè found that it behaved like a rare earth, not like rhenium, and since rare earths are typically prominent among fission fragments, it appeared that the activity was a rare-earth isotope.
At that time, the new 60-inch cyclotron began operating at Berkeley. It was the highest energy accelerator in the world, and the importance of Ernest Lawrence’s work in the field was recognised that same year, when he was awarded the 1939 Nobel Prize in Physics “for the invention and development of the cyclotron and for results obtained with it, especially with regard to artificial radioactive elements.” The 60-inch cyclotron and its 16-MeV deuterons offered new possibilities to McMillan's search for transuranium elements. During 1939, the fission process became better understood, and by early 1940 McMillan had become convinced that the new activity could not be the decay of a fission fragment and began a series of experiments with the new cyclotron. These confirmed that the 2.3-day activity could not be from fission and that the 23-minute uranium isotope uranium-239 was almost certainly genetically related to it. It appeared in fact that the beta decay of uranium-239 (Z=92) was producing atoms of a new element with Z=93! McMillan learned that the young Philip Abelson, who had returned to Berkeley for a short visit, had been working on the same problem. Together they were able to develop a key chemical procedure that allowed them to solve the final puzzle and conclusively establish that the 2.3-day activity actually was an isotope of element 93, the isotope 239. They proved that element 93 could be produced in very small quantities by a uranium reaction with neutrons that did not involve fission, and that it behaved chemically like uranium, and not like rhenium, as initially had been predicted.
At this point it became obvious why element 93 had evaded earlier seekers, since its chemistry was more like that of uranium than that of rhenium. This similarity to uranium led McMillan and Abelson to suggest that a new series of elements of closely related properties was starting here, similar to the phenomenon that occurs earlier in the periodic table with the rare earths. Because of this relation - and since uranium (element 92) had been named for the planet Uranus - McMillan proposed the name neptunium for element 93, lying beyond uranium, and plutonium for its daughter element 94, after the planet Pluto, which was, however, as yet unidentified. Neptunium became the first of the transuranium elements. A major barrier had broken down; Abelson and McMillan's chemical technique formed the basis of all the transuranic chemistry to follow.
The next step in the investigations was the identification of the element with atomic number 94. It was naturally supposed that the isotope 239 of element 94 would emit alpha particles, but a search for them in decayed samples of isotope 239 of element 93 showed an activity too weak for good experimentation. Soon thereafter, in bombarding uranium with deuterons McMillan and Abelson hoped to produce a different isotope of element 94, with a shorter half-life and thus emitting more energetic alpha particles. This hope proved to be justified, but they could not make the final identification of element 94, because in November 1940 McMillan was called away to participate in defence research at the Massachusetts Institute of Technology and became involved in the radar project.
In the meantime, Glenn Seaborg had asked one of his students, Arthur Wahl, to undertake an in-depth study of the chemistry of element 93 and after an agreement with McMillan, they continued the search for element 94. They bombarded uranium oxide with 16 MeV deuterons and were successful in isolating chemically the unstable element 93 fraction of the resulting products, which decayed by emitting a beta particle to yield element 94, with a mass number of 238. On the night of 23-24 February 1941, Seaborg, Wahl and Joseph Kennedy obtained final chemical proof of the discovery of the isotope 238 of element 94, a new transuranic element for which the name plutonium (Pu) had already been suggested by McMillan, who shared credit for the discovery.
Soon thereafter, with participation of Emilio Segrè, followed the discovery of the isotope plutonium-239. The small sample obtained was sufficient to reveal that it was susceptible to fission by bombardment with slow neutrons and therefore its production in substantial quantities was a matter of extreme importance to national defence. About this time, with World War II already in progress, the possible military use of a nuclear chain reaction was recognized, and the production of transuranium elements for military use began. In August 1942 Seaborg, along with a number of his colleagues, moved to the Metallurgical Laboratory of the University of Chicago (now the Argonne National Laboratory) to continue research on plutonium-239 and, in particular, to find a way to produce it in usable amounts for the production of an atomic bomb within what became the Plutonium Project. After the discovery of plutonium, Seaborg, also following McMillan's ideas, hypothesised that a new series of elements called the actinide series, akin to the lanthanide series (elements 58-71), was being produced, and that this new series began with thorium (Th), atomic number 90. Thereafter, discoveries were sought, and made, in accordance with this hypothesis. During the war, elements 95 and 96 were thus synthesised, identified, and respectively named americium (Am) and curium (Cm), after Pierre and Marie Curie, historical leading investigators in the field of radioactivity. These results were voluntarily withheld from publication until 1946, within the secrecy constrains of the Manhattan Project, the U.S. Government research project (1942-1945) that eventually produced the first atomic bombs.
For his discovery of neptunium with Abelson and of plutonium with Kennedy, Seaborg, and Wahl, McMillan was jointly awarded with Seaborg the Nobel Prize in chemistry in 1951. That same year, John Cockcroft and Ernest Walton received the Prize for “for their pioneer work on the transmutation of atomic nuclei by artificially accelerated atomic particles”.
After the war, the hunt for transuranium continued. So far more than 20 synthetic elements have been created. All are unstable, decaying with half-lives ranging from a year to a few milliseconds. Uranium, element 92, is the heaviest element known to exist naturally in detectable amounts on Earth. All elements with atomic numbers higher than that of uranium are radioactive with a half-life much shorter than the age of the Earth, so any atoms of these elements, if they ever were present at the Earth's formation, have long since decayed, but trace amounts form in some uranium-rich rocks. Many transuranium elements have been named after scientists: einsteinium (99), fermium (100), mendelevium (101), nobelium (102), lawrencium (103), rutherfordium (104), seaborgium (106), bohrium (107), meitnerium (109), roentgenium (111), copernicium (112), flerovium (114).
From Transuranium Research to Accelerators
After the war, while Seaborg continued to be involved in radiochemical work and discovered new transuranium elements, McMillan's thoughts reverted to the problem of accelerators, stimulated by the added experience and technology gained during the period of war research. Even before the 60-inch cyclotron produced its first beam in the spring of 1939, Lawrence had begun to think of the next step in the sequence of higher and higher energy accelerators. But the new planned 184-inch cyclotron was never assembled, because the magnet was used by Lawrence at the Oak Ridge pilot plant for the electromagnetic method of separation of the fissile uranium-235. Now, in 1945, the cyclotron would be completed. Lawrence and his associates hoped it would accelerate particles to 100 million eV, which was a sort of barrier beyond which conventional cyclotrons could not go because of technical difficulties related to the acceleration of particles.
In cyclic accelerators, a charged particle in a uniform constant magnetic field B describes a circular path of r radius such that Br=vm/e, where m/e is the mass to charge ratio of the particle and v is the velocity. The time per revolution will thus be t= 2πBm/e. This is essentially a constant in the nonrelativistic range; hence the repeated application of an alternating electric field of frequency 1/t between two “D”-shaped electrodes (dees) will give an incremental acceleration each time the particle comes around in its circular orbit in the proper phase. Continuous particle acceleration thus requires a synchronism (resonance) between the particle motion and changes in the accelerating field during each transit through the accelerating gap. To achieve this, the voltage frequency must match the particle's cyclotron resonance frequency, which is inversely proportional to the relativistic mass of the particle. If then the particle is accelerated until its velocity is half the velocity of light, the mass is 15 percent greater than the rest mass and the time t is 15 percent greater than for the first turn. Under these conditions the particles would get out of phase with the accelerating field and would slip out of the acceleration mode. Therefore, a conventional cyclotron features a limiting energy beyond which further acceleration is impossible.
The problem of circumventing the energy limit was solved by McMillan, who had already designed some power supplies for the machine. One night in June/July 1945, his mind returned to the concept of resonance acceleration and he wondered “if there were only some way to keep the motion of the particles in step with the alternating electric field that was pushing them along,” when he suddenly realised that the motion had a natural tendency to lock into step with the accelerating field, if certain simple conditions were satisfied.
Supposing that a charged particle in the extreme relativistic energy range is pursuing a circular path of radius r in a uniform magnetic field. If a voltage of the proper radio frequency is applied with such a phase that the voltage goes though zero as the particle crosses the accelerating gap, there will be no change in its speed, energy or mass. Another particle, arriving at the gap earlier will acquire a greater energy, and will hence follow a longer circular path and be later in crossing the accelerating gap the next time. Conversely, a particle arriving too late would be decelerated, pursuing a shorter path, and would catch up in time. The particle, if disturbed in phase, then oscillates about the stable phase, where it gains no energy in crossing the gap in such a way that it will try to fall in line with the well-behaved one. This tendency to get together on the equilibrium orbit is so strong that if the guiding magnetic field or the frequency of the oscillation period of the electric accelerating field or both are slowly changed in the proper direction, such changes in the applied forces would so stabilise the timing relations that the particles would, after following such more or less arbitrary variations, stabilise in a new equilibrium orbit, corresponding to a higher particle energy. The stability will hold for the phase such that just enough energy is gained during each revolution to keep step with the changing field or frequency. This process can be continued as slowly or rapidly as the designer wishes, until the particles are boosted to the desired energy.
McMillan felt “like the inventor in a cartoon with a lightbulb flashing on over his head.” He soon had a name for the locking-in phenomenon, which he called “phase stability” because the word “phase” was used to describe the timing relation.
He also had a name for the accelerator that would use that principle, the “synchrotron,” and he prepared a short report describing the idea: “This is a device for the acceleration of particles to high energies. It is essentially a cyclotron in which either the magnetic field or the frequency is varied during the acceleration, and in which the phase of the particles with respect to the high energy electric field automatically adjusts itself to the proper value for acceleration.”
McMillan published his discovery in the Physical Review in September 1945. Only at that time, the U.S. science community became aware that the Soviet scientist Vladimir Veksler had independently discovered the same principle, which revolutionised accelerator design and construction throughout the world.
The principle of “phase stability” became the fundamental ingredient of the synchrotron, but could be applied to many types of high-energy accelerators. McMillan immediately moved to implement his ideas; the original plans for the 184-inch cyclotron at Berkeley were modified, turning it into a “synchro-cyclotron,” which produced its first beam of deuterons at 195 MeV on November 1, 1946. Elements 103, 104 and 105 were discovered and new techniques in diagnosing disease with radioisotopes were developed, later used around the world. The early years of the experimental program also included the first accelerator production of pions and the discovery of the neutral π-meson. A new era in high-energy particle physics began.
The phase-stability principle exerted a revolutionising effect on the development of accelerator engineering. The success of the new machines based on the principle of phase stability provided the impetus for a new stage of accelerator building at Berkeley, the Bevatron. A new generation of big accelerators was also built in Russia and all over the United States, as well as in France, Italy, at the new European laboratory at CERN, in the United Kingdom, and in Australia.
All accelerated particles, typically electrons, are a source of electromagnetic radiation, first observed in synchrotrons. Synchrotron light deliberately produced by storage rings and other specialised particle accelerators can be used as a tool for many types of scientific research and technical purposes. The major applications of synchrotron light are in condensed matter physics, materials science, biology and medicine.
For their discovery, McMillan and Veksler eventually were chosen to share the Atoms-for-Peace Prize in 1963. Following Ernest Lawrence's untimely death in 1958, McMillan had been appointed director of the Radiation Laboratory. Under his leadership, it was renamed Lawrence Berkeley Laboratory and became a leading international centre for nuclear and high-energy particle physics. The principle of phase stability is one of the fundamentals on which the large accelerators of today are based. They are miles in circumference, have energies measured in TeV, and are used at the forefront of particle research.
Bibliography
Jackson, J. and Panofsky, W.K.H. (1996) Edwin Mattison McMillan (1907-1991). National Academy of Sciences. Biographical Memoirs 69: 215-242.
Seaborg, G. T. (1993) Edwin Mattison McMillan (18 September 1907 - 7 September 1991) Proceedings of the American Philosophical Society 137: 286-291
Lofgren E. J. (1993) Edwin McMillan (1907-1991). In K. J. Laylin (ed.), Nobel Laureates in Chemistry. 1901-1992. American Chemical Society and the Chemical Heritage Foundation, pp. 338-343
McMillan, E. (1979) Early History of Particle Accelerators. In R. H. Stuewer (ed) Nuclear Physics in Retrospect. University of Minnesota Press, pp. 113-155
McMillan, E. (1959) History of the Cyclotron, Part 2. Physics Today 12: 18-34
McMillan, E. (1984) A history of the synchrotron. Physics Today 37: 31-37
Seaborg, G. T. and Segrè, E. (1947) The trans-uranium elements. Nature 159(4052): 863-865
Interview with Dr. Edwin McMillan by Charles Weiner, June 1, 1972 (http://www.aip.org/history/ohilist/4773_1.html)