Prof. Dr. Leon M. Lederman > Research Profile
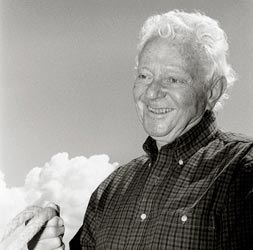
by Roberto Lalli
Leon M. Lederman
Nobel Prize in Physics 1988
Lederman’s Early Work with Particle Accelerators
Leon M. Lederman was born in New York City on 15 July 1922, as the second son of Russian-Jewish immigrants. Lederman majored in chemistry at the City College of New York in 1943. During his undergraduate years, he became fascinated by the logical rigour of physics and was influenced by future physicists such as Isaac Halpern and Martin J. Klein. After World War II, Lederman decided to pursue his interest in physics entering the Graduate School of Physics at Columbia University, New York City, in 1946. Chaired by I. I. Rabi, the Columbia physics department was rapidly assuming a leading role in the US post-World War II nuclear research. Following the war-related experience at the MIT Radiation Laboratory, Rabi was building a research group made up of both experimentalists and theorists, who were willing to work together in order to set up and interpret new experiments aimed at discovering the properties of nuclei and sub-nuclear particles. In addition, Columbia was at the forefront on the development of particle accelerators, which in the early 1950s replaced cosmic-ray observations as the main mean for the empirical study of particle properties. In 1947, Columbia began operating the Nevis Laboratory, which at the end of the 1940s housed the most powerful synchrotron of the world. The close Brookhaven National laboratory (BNL) was also established in 1947, with the primary purpose to serve the universities of the East Coast. In 1952, the proton synchrotron of the BNL—the Cosmotron—reached its maximum strength, accelerating protons up to a kinetic energy of 3.3 GeV, thus becoming the first accelerator operating in the GeV range.
Lederman graduated in physics in 1948 and continued to pursue his PhD studies at Columbia. He worked at the recently built 385 MeV Synchrocyclotron of the Nevis Laboratory under the direction of the American nuclear physicist Eugene T. Booth. The group of researchers headed by Booth was investigating the properties of the recently discovered π-meson (or pion). Through cosmic-ray observations, a research team composed by C. Lattes, G. Occhialini, C. Powell and others had provided clear evidence that there existed two different particles with similar mass, but very different decay properties. The discovered particles—the π-mesons—interacted strongly with matter and decayed rapidly into µ-mesons (or muons), which in turn decayed only weakly. Lederman was pursuing his PhD in a period marked by a deep and rapid shift in experimental practice from cosmic ray observations to laboratory accelerators. In accelerators, pions could be produced, along with other charged particles, by accelerating protons against a target. Lederman’s PhD task was to set up the detector for these charged particles: a 16-inch Wilson cloud chamber under a magnetic field of 4040 gauss.
After earning his PhD in 1951, Lederman continued to work at the Nevis Laboratory for the next 28 years, becoming its director in 1961. In the 1950s, Lederman focused especially on the study of a new type of particles, discovered in 1947 in cosmic-ray observations. Because of their peculiar behaviour, these particles were christened ‘strange’ particles. After their discovery, further observations showed that there were many such unexpected particles, differing in mass, lifetime and decay products. In a conference held at Rochester in 1954, physicists listed 13 different hypothetical particles—some of them being different decays of what are now understood as the same particle. Among the various partial classifications, the most successful was to divide the strange particles into K-mesons (if their mass was between the pion’s and the proton’s mass) and hyperons (if their mass was between the neutron’s and the deuteron’s mass).
In 1954, the theoretical physicists M. Gell-Mann and A. Pais proposed a theoretical classification of the strange particles, which included the so-called neutral K-particle complex. Charge conjugation invariance implied that the neutral K-meson (or kaon) and its anti-particle mixed, giving rise to two states with well-defined decay properties and lifetimes. Theoretical investigations suggested that one of these two states had a lifetime much greater than the other. For this reason these two states were labelled Kl—for the long-lived neutral kaon—and Ks—for the short-lived neutral kaon. By 1955, only the short-lived Ks had been experimentally observed through the bi-pion decay Ks => π++ π-.
A seminar given by Pais at Columbia in 1955 and some remarks by O. Piccioni prompted Lederman and his group to perform an experiment aimed at testing the existence of the Kl, employing the same cloud chamber they were already employing for the analysis of strange particles at the BNL Cosmotron. By mid-1956, Lederman and his collaborators had provided convincing evidence for the existence of the Kl and established its three-body decay into, e.g., πµv, πev, or 3π (where v means neutrino, and e electron). This discovery was the last one made with a Wilson chamber, which was rapidly being replaced by the more efficient bubble chamber, invented in 1952 by D. Glaser.
The year following the experimental discovery of the long-lived neutral K-meson, Lederman, along with R. Galwin and the doctoral student M. Weinrich, confirmed that parity and charge conjugation are violated in weak interactions. In 1956, the Chinese-American theoretical physicists T. D. Lee and C. N. Yang had suggested parity non-conservation in weak interaction and had discussed a series of hypothetical experimental tests of this hypothesis. After the Columbia researcher C. S. Wu had first provided experimental evidence of non-conservation of parity in beta decays, the group led by Lederman rapidly set up an experiment to test the violation of parity in the decay π → µ → e. In the course of a single weekend the experiment was completed, becoming the third confirmation of parity non-conservation in weak interactions. The evidence provided by the various experimental groups was so strong that Lee and Yang received the Physics Nobel Prize in 1957, only one year after they had put forward their hypothesis. The Galwin-Lederman-Weinrich experiment also furnished an early measurement of the muon g-2 factor. The g-2 factor is the difference between the empirical gyromagnetic ratio of the muon and the theoretical value for a spin ½ particle according to the relativistic Dirac equation (the analogue of the anomalous magnetic moment of the electron discovered in 1947). In 1957, Lederman and his co-workers found that the muon gyromagnetic ratio was 2.00 ± 0.10 (i.e., the muon g-2 factor was very close to zero), providing strong support to the hypothesis that the µ-meson was a spin ½ particle.
In 1958, Leon Lederman took his first sabbatical at CERN where he organized a research group to work at the g-2 experiment. This CERN program would continue for about 19 years. It was the first of several collaborations of Lederman with CERN, which endured through the mid-1970s.
The First High-Energy Neutrino Experiment
In 1960, the Columbia researcher Melvin Schwartz proposed a method to produce a beam of high-energy neutrinos at the accelerator under construction at the BNL: the Alternating Gradient Synchroton (AGS). Schwartz’s reasoning went as follows. The collision between the high-intensity high-energy beam of protons and the target nuclei copiously produced pions, which in turn rapidly decayed into muons and neutrinos. Neutrinos interact only weakly with matter, so physicists should look for weak reactions triggered by the neutrinos, such as the inverse of beta decay or of µ-decay. Since the AGS was planned to produce a high number of high-energy neutrinos, a very massive detector could be able to detect a sufficient number of these neutrino interactions. The idea proposed by Schwartz, and simultaneously by B. Pontecorvo, seemed promising, because it allowed for an in-depth experimental study of weak interactions.
In May 1960, a Columbia team including Lederman, Schwartz, J. Steinberger and others submitted a proposal to the BNL in order to perform such an experiment at the AGS. The AGS was completed in July 1960, when it reached the energy of 33 GeV—then the highest energy reached by particle accelerators. In September 1960, the team decided to replace the bubble chamber with the recently invented spark chamber. The spark chamber was much more effective for the type of experiment the group had in mind because it allowed for a clear distinction between electrons and muons. The final design was much more complex than the one initially proposed by Schwartz, because it had to take into account all the possible background effects.
The experiments had three different aims. First, it introduced a new experimental method, showing that the high-energy neutrino beams might effectively be produced in laboratories and their reactions analysed. Second, it could be employed for the detection of the W-bosons that were supposed to mediate weak interactions. Third, the physicists wanted to test the theoretical hypothesis that there existed two different kinds of neutrinos, related respectively to the electron (or positron) and muon (or anti-muon). The high-energy neutrinos produced at the AGS were by-products of pions decaying into muons. If the outgoing particles detected by the spark chamber were only muons, it would have meant that there existed two different types of neutrino. If both electrons and muons were produced, the experiment would have shown that there existed only one type of neutrino.
On June 15, 1962, the group of researchers submitted their result to Physical Review Letters, rapidly published with the title “Observations of High-Energy Neutrino Reactions and the Existence of Two Kinds of Neutrinos.” The experiment, they claimed, offered clear evidence that only muons were produced by the high-energy neutrino interactions. As a consequence, the two-neutrino hypothesis gained momentum and was rapidly accepted by the physics community. A second noteworthy outcome of this groundbreaking experiment was that, from then onward, neutrino beams could be employed to study the properties of weak interactions. In 1988, Steinberger, Lederman, and Schwartz were awarded the Nobel Prize in Physics “for the neutrino beam method and the demonstration of the doublet structure of the leptons through the discovery of the muon neutrino.”
The third target of the experiment—the discovery of the W-bosons—was not successful, and Lederman continued to pursue the search for these particles in the following years.
Looking for the W Particles and Finding the Bottom Quark
The discovery of the muon-type neutrino prompted experimental physicists to increase their efforts to study the properties of weak interactions in particle accelerators. The most accepted theoretical model for weak interaction was the V-A theory put forward in 1957. The universal V-A theory described the weak interaction as a combination of vector and axial vector currents. Weak interactions were supposed to be mediated by charged vector bosons usually referred to as W+ and W-. After the discovery of the muon-type neutrino, the theory predicted that the Ws could decay in muons plus muon-type neutrinos—just like pions and kaons. Since the quantity of W-particles produced in hadron collisions was expected to be just one millionth of the number of pions and kaons, experimenters were striving to elaborate strategies to eliminate the background and observe particles that could actually be considered by-products of W-decays. One of the tactics was to observe muons at large angles with respect to the line of flight of the accelerated protons, as pions and kaons were expected to run primarily along the direction of protons’ flight. In contrast, the large mass of the W-particles could give rise to muons travelling at large transverse momentum.
After failed attempts to detect the W particles in this way both at the BNL and CERN, Lederman and a Columbia group working at the BNL devised an experiment aimed at detecting the production of lepton pairs from hadron collisions. The technical name of the experiment was ‘dimuon experiment’; the central reaction was p + nucleus => µ+ + µ- + anything. The rationale underlying this decision was that some theoretical schemes predicted neutral vector bosons or high energy virtual photons, which could decay to muon-antimuon pairs.
This experiment began in 1967. While it was conducted, a different experiment provided strong support to the so-called parton-quark model. In 1969, experiments performed at the Stanford Linear Accelerator (SLAC) showed that the deep inelastic scattering of high-energy electrons accelerated against protons and deuterium nuclei were compatible with a description of the nucleons as composed of quasi-free point-like constituents. Many theoretical interpretations of the deep inelasting scattering data were provided, but the idea that nucleons were composed of smaller particles and that these particles had properties similar to the quarks earlier proposed by Gell-Mann rapidly gained momentum.
The data of the Columbia-BNL dimuon experiment showed a steeply decreasing continuum and a sort of shoulder, understood as a possible resonance (see fig. 1). In 1970, the theoretical physicists S. Drell and T.-M. Yan proposed that the steeper part of fig.1 was a confirmation of the parton model, interpreting the virtual photon decaying into the muon pair as produced by an annihilation of a quark and an anti-quark of the interacting hadrons.
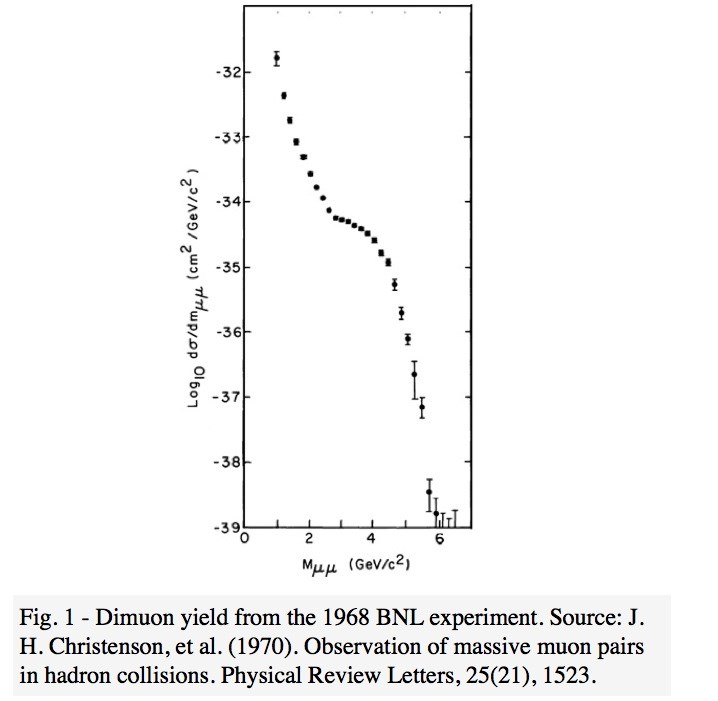
Since the dimuon experiment was considered of interest as a test of the parton model and since the Columbia team had demonstrated that it was technically feasible, it was planned to increase the energy of the lepton pairs produced in hadron collisions. In 1971-72, Lederman led an experiment at the Intersecting Storage Rings (ISR) at CERN—the first hadron collider of the world—to study the production of electron pairs instead of muon pairs. The first aim of these kinds of experiments was to understand the strange shoulder-like shape (fig. 1) around 3,5 GeV that was not explainable by the parton model. The CERN-Columbia-Rockfeller team was, however, unable to shed light on the resonance found in the previous experiment because of an unexpected background of high transverse momentum hadrons. The detected variation from the expected exponential fall-off of hadrons with large transverse momentum was shortly interpreted as a confirmation of the parton model, and it later became a support of quantum chromodynamics when that theory was formulated in 1973.
Employing a higher mass resolution, a group led by S. Ting made an analogous experiment on dielectron production at the BNL finding a clear peak at 3.1 GeV. The data were a strong indication that there existed a neutral particle of this mass, soon baptised J particle. A different kind of experiment conducted at the SLAC under the direction of B. Richter simultaneously produced evidence for a particle of this mass, which was called Psi. Soon after, the J/Psi particle was understood as composed of the hypothetical charm quark. This discovery was instrumental for increasing of the consensus around the quantum field theories of electroweak interaction and of the strong interaction, which would later be called the Standard Model of particle physics. Since the discovery was made in November 1974, the period is usually referred to as the November revolution. “For their pioneering work in the discovery of a heavy elementary particle of a new kind,” Ting and Richter were awarded the 1976 Nobel Prize in Physics.
In the meanwhile, the promising results on dilepton experiments both at the BNL and CERN encouraged Lederman to plan similar kinds of experiments at the Fermilab, then under construction. The initial aim of the group was again to make sense of the shoulder found in the original dimuon experiment. Since the discovery of the J/Psi particle in 1974 had made this goal out-dated, Lederman’s group decided to analyse lepton pairs produced by more energetic virtual photons. In 1977, several improvements concerning the detector elements, the target material, and the shielding, allowed the team to increase the number of detected lepton pairs as well as to drastically reduce the background. In particular, the newly developed multiwire proportional chamber was employed as detector. Moreover, the group worked with the Fermilab synchrotron, which could accelerate protons up to 500 GeV. In the spring of 1977, the group had detected about 7000 electron pairs. The data collected during this experiment—called the E288 experiment—allowed the experimenters to state that a new particle of mass near 9,5 GeV existed (see fig.2). The particle was named upsilon and it was soon understood as composed of a new massive quark.
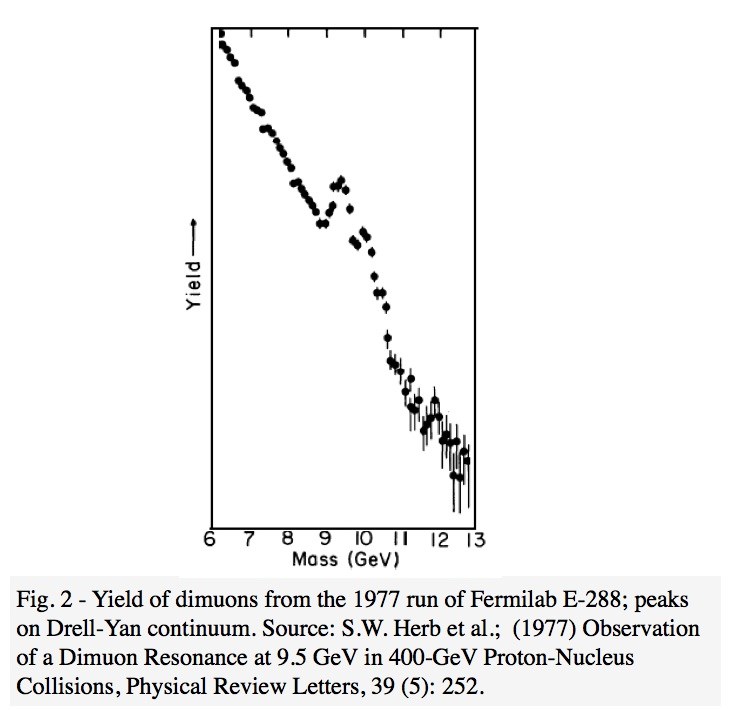
Experiments performed from 1974 to 1977 at the colliding ring of the SLAC had convinced the experimenters that they had detected a new lepton of mass much greater than that of the muon. The discovery of the tau-lepton—as the particle was christened—prompted several theoretical analyses promoting the idea of a three-family structure for both leptons and quarks. The theoretical physicists M. Kobayashi and T. Maskawa explicitly put forward this idea, by predicting that the third family of quarks should be made of a doublet of a -1/3 electrical charge quark (later called bottom quark) and a +2/3 electrical charge quark (later called top quark). Theoretical investigations also predicted that the members of the third family of quarks should be much more massive that the quarks of the first two families.
While the group led by Lederman did not make any inference concerning the theoretical meaning of their empirical findings, theorists soon interpreted the upsilon as composed of one of the two quarks of the hypothetical third family. Further experimental data provided by Lederman and his co-workers confirmed this hypothesis and clarified the properties of such a new quark. The empirical data provided strong evidence that its charge was -1/3 and thus was the bottom quark, predicted by Kobayashi and Maskawa. Soon after, experiments made at high-energy electron-positron colliders confirmed the existence of the upsilon. These experiments also confirmed the initial data provided by the Fermilab experiments and its theoretical interpretations. “For the discovery of the origin of the broken symmetry which predicts the existence of at least three families of quarks in nature,” Kobayashi and Maskawa were awarded half of the 2008 Nobel Prize in Physics, the other half being awarded to Yoichiro Nambu “for the discovery of the mechanism of spontaneous broken symmetry in subatomic physics.”
Striving for the Construction of New Laboratories
After the discovery of the muon-type neutrino, in 1963 Lederman promoted the idea of a new laboratory that eventually became the Fermi National Accelerator Laboratory in Batavia, Illinois (Fermilab) founded in 1967. In 1979, Lederman became the director of the Fermilab leading the plans for the construction of the Tetravon—the first particle accelerator to reach the kinetic energy of 1TeV. After the Tetravon began operating in 1983 it became the most powerful accelerator of the world until 2009, when the CERN Large Hadron Collider began operations. Among the various achievements made with the Tetravon, the discovery of the top quark in 1995 stands out. Lederman stepped down before this discovery, becoming Director Emeritus in 1989, the year after he had gained the Nobel Prize.
In the 1980s, Lederman played a key role as proposer and supporter of the Superconducting Super Collider (SSC) in the United States—which was planned to be the largest and most powerful accelerator in the world. He also testified before the U.S. Congress in favour of such an endeavour. In 1993, he published the book “The God Particle: If the Universe Is the Answer, What is the Question?” in which he tried to increase the consensus for the need of the new particle accelerator. That very same year, after 2 billion dollars had already been invested in the project, the U.S. Congress eventually dismissed it, provoking a bitter reaction from Lederman and other physicists who had supported the project.
Since his retirement as the director of the Fermilab, Lederman has given much attention to science education and founded the Illinois Mathematics and Science Academy, the first state wide residence public school for gifted children, as well as the Teacher's Academy of Mathematics and Science in Chicago. Currently, he holds the chair of Pritzker Professor of Science at the Illinois Institute of Technology, Chicago.
Bibliography
Fitch V. L., & Rosner L. (1995) Elementary Particle Physics in the Second Half of the Twentieth Century. In Brown, L., Pippard, B., & Pais, A. (Eds.). Twentieth century physics (Vol. 2). AIP, New York, pp. 635-794.
Hoddeson, L. Brown, L. M., Riordan, M. & Dresden, M., (1995) The rise of the Standard Model: Particle Physics in the 1960s and the 1970s. Cambridge University Press, Cambridge.
Lederman, L. M. (1989) Observations in particle physics from two neutrinos to the Standard Model. Reviews of Modern Physics, 61(3), pp. 547-560.
Lederman, L. (1991) Leon M. Lederman - Biographical". Nobelprize.org. Nobel Media AB 2013. Accessed 5 April 2014. http://www.nobelprize.org/nobel_prizes/physics/laureates/1988/lederman-bio.html
Lederman, L. (1993) The God Particle: If the Universe Is the Answer, What is the Question? Houghton Mifflin, Boston.
Pais, A. (1986) Inward Bound Of Matter And Forces In The Physical World. Clarendon Press, Oxford.
Pickering, A. (1999) Constructing quarks: A sociological history of particle physics. University of Chicago Press, Chicago.