Prof. Dr. John Cowdery Kendrew > Research Profile
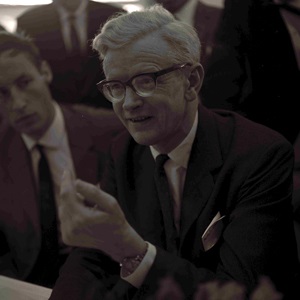
by Luisa Bonolis
John C. Kendrew
Nobel Prize in Chemistry 1962 together with Max F. Perutz
"for their studies of the structures of globular proteins".
Going into molecular biology
John Kendrew was born in Oxford, where his father was a noted geographer and reader in climatology at the University. At school he was an excellent student, especially outstanding in chemistry. By the time he graduated from Clifton College in Bristol he had already decided upon a scientific career. In 1936, he won a Major Scholarship to Trinity College, Cambridge, where he attended courses by Ernest Rutherford and J. J. Thomson and became interested in proteins, following his supervisor in biochemistry F. J. W. Roughton. Under the influence of his mother, Evelyn May Graham Sandberg, an art historian, he also developed a strong passion for music, archaeology, and architecture, which he maintained for the rest of his life.
In June 1939, Kendrew graduated in chemistry with a first-class honours degree, but his early researches were broken off because of the outbreak of World War II. He was recruited into the Telecommunications Research Establishment, where he worked on oscillators and early radar applications. After being appointed as a junior research officer to Air Ministry Research, he became deeply involved in radar research. In 1944 he became scientific adviser to the Allied air commander in chief, based in Southeast Asia.
During the later part of World War II, Kendrew became acquainted with the British chemist John Bernal, who discussed with him the convergence of physics and biology and explained to him that to understand life it was necessary to understand the structure of proteins. Almost all chemical reactions that take place in cells are catalysed by enzymes, and all enzymes are proteins; an individual cell contains perhaps 1000 different kinds of enzymes, each catalysing a different and specific reaction. Proteins have many other important functions, being constituents of bones, muscles and tendons, of blood, of hair and skin and membranes. Bernal explained to Kendrew the great and challenging possibility of determining protein structures by means of X-ray analysis, a technique that can be used to determine the three-dimensional molecular structure of substances that can be wholly or partly crystallized. During the 1930s, Bernal had undertaken the earliest crystallographic studies of proteins with Dorothy Crowfoot Hodgkin and with Max Perutz. Hodgkin later moved to Oxford, and together with her colleagues in Cambridge she became one of the founders of protein crystallography, making an outstanding contribution to structural biology by solving the crystal structures of cholesterol, penicillin, vitamin B12, and, finally, insulin. She was awarded the Nobel Prize for Chemistry in 1964 “for her determinations by X-ray techniques of the structures of important biochemical substances”.
Bernal suggested that Kendrew should contact Perutz at the Cavendish on returning to England. In March 1945, in going back home from India via Australia, the USA and Canada, Kendrew visited Linus Pauling, one of the most influential chemists in history, one of the founders of the field of quantum chemistry, and a Nobel laureate for chemistry in 1954. Pauling was also a very skilled crystallographer and one of the fathers of molecular biology. He, too, told Kendrew that protein structure was of central importance in biology, so that Kendrew's interest in protein crystallography was further amplified and he became determined to work on the molecular structure of proteins.
The fathers of X-ray crystallography
In the autumn of 1945, Kendrew visited Max Perutz in Cambridge and from 1946 he began working with him at the Cavendish Laboratory. At that time, Perutz had resumed his earlier investigations into the molecular structure of globular proteins using the technique of X-ray crystallography. This method of research stemmed from a discovery made in Spring 1912 by Max von Laue in Munich. Von Laue had expressed the idea that the spacings of a crystal lattice would be of the right size to cause the diffraction of X-rays, supposing them to be very short electromagnetic waves. The phenomenon of interference was well known in optics using ordinary light. Light waves hitting regularly spaced objects (e.g., equally spaced scratches in a glass plate) enhance each other in certain directions and work against each other in other directions. In this manner, an interference pattern or diffraction pattern is produced. To obtain interference, the size of the wavelength has to be of the same order of magnitude as the distance between the diffracting. Von Laue's hypothesis had been brilliantly confirmed by Walter Friedrich and Paul Knipping and it ended a decade and a half of unsystematic searching for an interference effect in X rays. A few months later, Lawrence Bragg, then a student at Cambridge, visualised this lattice structure as being constructed from a series of sheets of atoms, laid one on top of another, and conceptualised the effect as that of the reflection of X rays by the successive parallel crystal planes, acting like a mirror. Lawrence Bragg also proposed that the ratio between the wavelengths and the distances of the planes from each other could be calculated by a simple but powerful equation relating the wavelength of incident wave, the spacing of the planes in the atomic lattice and the angle between the incident ray and the scattering planes (the glancing angle) at which constructive interference was observed. This expression became universally known in the community of crystallographers as “Bragg's law.” Bragg’s law (and the notion of reflection) were particularly important in the early development of X-ray crystallography, because it rendered the process of diffraction easier to visualize and simplified calculations.
These early investigations were performed by Lawrence Bragg together with his father Henry Bragg. They built an X-ray spectrometer with which they analysed the structure of several salts and small molecules, establishing fundamental mathematical relationships between an X-ray diffraction pattern and the dimensional arrangement of atoms in a crystal that produced the pattern. In their early work, the Braggs made a series of approximations in order to unravel the complex tangle which the reflexions from some crystal represented. What Lawrence Bragg did was to calculate the expected intensities and to compare these values with the observed intensities. From the starting positions of the atoms (based mainly on packing considerations), the atoms were moved until the calculated and observed structure factors were in the best possible agreement. This “trial-and-error” method for structure determination worked because the compounds were simple and the calculation method was very sensitive to the positions of the atoms.
The Braggs reduced X-ray analysis of simple materials to a standard procedure by 1914, and both headed a major school of X-ray crystallography between the wars. Their findings created the new science of X-ray crystallography, making it possible to determine molecular structures from the crystal form of a compound.
Von Laue was awarded the 1914 Nobel Prize for Physics for his breakthrough discovery, while the Braggs, father and son, were jointly awarded the 1915 Prize for Physics. “for their services in the analysis of crystal structure by means of X-rays.''
In the years since modern crystallography dawned with X-ray diffraction experiments on crystals, the discipline has informed almost every branch of science, leading to further developments in spectroscopy and solid-state physics and providing a means to understand the structure of highly complex molecules and materials, eventually giving rise to the field of molecular biology.
However, as researchers began to use the Bragg's X-ray crystallography method to identify increasingly complex structures, they soon discovered its limits. Each type of crystal has its own characteristic arrangement of atoms and so will produce its own specific X-ray pattern, the features of which can be unambiguously, if tediously, predicted by calculation if the structure of the crystal is known. But X-ray analysis involves the reverse calculation: Given the X-ray pattern, what is the crystal structure that must have produced it? Each spot on a diffraction pattern provides information only about the intensity of the X-ray waves that have been deflected off the atoms in the crystal into the path of that spot. Ideally researchers also needed to know which particular point in the undulating cycle of movement from one wave peak to the next - known as the phase of the wave - these X-ray waves are at when they hit the film and form each spot in the diffraction pattern. In the case of simple molecules containing only tens of atoms, the diffraction pattern and wave intensity information was sufficient to identify a structure, with the help of a technique devised by the French mathematician Joseph Fourier in the 19th century, which breaks down complex waveforms into their constituent waves. Although it was a painstaking task, researchers in the early years of crystallography could almost always rely on Fourier synthesis, together with a healthy dose of trial-and-error and educated guesses, to hit upon the right structure of these compounds. However, larger molecules with thousands of atoms, like haemoglobin, posed a much greater problem. The number of reflections and interactions that occur within crystals of this size is so complex that to translate X-ray patterns into molecular structures requires more than just knowledge about the intensity of waves. To have any chance of deducing the structure, researchers needed to find a way of knowing what phase the X-ray waves are at when they form each spot in the diffraction pattern. This information could be calculated by knowing the phase angle, the distance between a point on a wave and a specified reference point. However, this vital piece of information, couldn't be directly obtained from the Fourier synthesis, or indeed any other method and remained missing in the process of making protein structure determinations possible. Researchers referred to this missing information as 'the phase problem', and at the time solving this was the ultimate goal for anyone interested in trying to determine protein structures from X-ray crystallography. Finding a solution to the phase problem in protein crystallography required a lot of time.
Without this missing phase information, researchers turned to another method that tried to get around the problem. Lindo Patterson devised a variation of Fourier synthesis in the 1930s, developing a method that partially avoided the phase problem by creating a contour map that could be used to define the distances between atoms in a crystal, knowing only the position and intensity data in an X-ray photograph. The upside of these Patterson maps was that you didn't need to know the phases of the reflections, but the downside was that creating a Patterson map was a hugely time-consuming task even for simple compounds, involving hundreds of calculations for each spot in a diffraction pattern. In addition, Patterson maps were not necessarily guaranteed to be successful with more complex compounds. Many workers felt that it was not satisfactory as a direct means of solving protein structures.
The structure of proteins
The Braggs led the field of X-ray crystallography for several decades, encouraging a new generation of researchers, including John Bernal and William Astbury, both pioneers of molecular biology. In 1934 Bernal was the first crystallographer to obtain clear images of X-ray diffraction by a protein, pepsin, after discovering that the crystals had to be kept in their mother liquor, becoming the founder of protein crystallography. Since the late 1930s, Max Perutz had interested Lawrence Bragg in the crystallographic analysis of the complex globular proteins. This research was resumed after the war, and Bragg organised the research effort, found support for the project, and assembled a team to tackle these new problems. In his activity as an outstanding scientific organiser, Bragg promoted the establishment of specific laboratories for research into the molecular structure of biological systems. The Molecular Biology Unit at Cavendish Laboratory in Cambridge led by Perutz attracted researchers who felt that the new field of molecular biology had great promise. Among them were Francis Crick and James Watson who proposed in 1953 a double helix structure for the polynucleotide chain configuration of DNA, an achievement for which they shared the Nobel Prize in Physiology or Medicine with Maurice Wilkins in 1962.
Initially Kendrew worked with Bragg on the comparison of foetal and adult haemoglobins. To understand the behaviour of a living cell, it is necessary first to find out how so wide a variety of functions taking place within the cell can be performed by molecules all made up for the most part of the same basic units. These units are the amino acids, of which there are about 20 different types, joined together to form the long molecular chains known as polypeptides. Following the classic researches on the insulin molecule by Frederick Sanger, who was later awarded the 1958 Nobel Prize for Chemistry, several groups of investigators were able to discover the order in which the amino acids are arranged in the polypeptide chains of a number of proteins. All protein molecules contain polypeptide chains and some of them contain no other constituents; in others there is an additional group of a different kind. For example, the haemoglobin in red blood corpuscles contains four polypeptide chains and four so-called haeme groups: flat assemblages of atoms with an iron atom at the centre. The function of the haeme group is to combine reversibly with a molecule of oxygen, which is then carried by the blood from the lungs to the tissues. With Perutz, Kendrew formulated a helical model for polypeptides that anticipated Linus Pauling's publication of his alpha helix. The alpha helix later revealed one of the most common secondary structures in proteins.
However, a polypeptide chain of perhaps hundreds of links can be arranged in space in an almost infinite number of ways. Chemical methods give only the order of the links; equally important is their arrangement in space, the way in which particular side chains form crosslinks to bind the whole structure together into a nearly spherical object (as most proteins are known to be). Of equal importance is the way in which certain key amino acid units, perhaps lying far apart in the sequence, are brought together by the three-dimensional folding to form a particular constellation - the so-called active site of the molecule - that enables the protein to perform its special functions. How is it possible to discover the three-dimensional arrangement of a molecule as complicated as a protein? The key to the problem is that many proteins can be persuaded to crystallize, and often their crystals are as regular and as nearly perfect in shape as the crystals of simpler compounds. Crystallization actually implies a regular three-dimensional array of identical molecules. The existence of protein crystals means, in fact, that proteins do have a definite three-dimensional structure to solve. And the most powerful techniques for studying the structures of crystals are those of X-ray crystallography.
Protein molecules, which contain, at minimum, thousands of atoms, have enormously convoluted and irregular formations that are extremely difficult to elucidate. In the 1930s Bernal, Dorothy Hodgkin and Perutz performed the earliest crystallographic studies of proteins at Cambridge’s Cavendish Laboratory; however, the intricacies of three-dimensional structure of proteins were too complex for analysis by conventional X-ray crystallography, and calculations performed by slide-rules and electric calculators were far too slow. It was not until the late 1940s, when Kendrew joined the Cavendish Laboratory as a graduate student, that new and more sophisticated tools emerged, which could be used to attack the problem.
Haemoglobin and Myoglobin
In 1947 Kendrew followed Perutz to the Unit for Molecular Biology, established that year at the Cavendish Laboratory by the Medical Research Council. Perutz continued his study of haemoglobin, the oxygen-transport protein, while Kendrew, after receiving his doctorate in 1949, shifted his attention to the structure of myoglobin, a small, bright red protein contained within the cells of muscular tissue, which acts as a temporary storehouse for the oxygen brought by the haemoglobin in the blood. It gives meat much of its red colour. Complex though it is, it is one of the smallest protein molecules, some of which are 10 or even 100 times larger. Although considerably simpler in structure than haemoglobin, being a quarter its size and consisting of a single polypeptide chain, myoglobin posed a formidable challenge, consisting of some 150 amino acids units – for a total of about 2600 atoms - together with a single haeme group.
The question then was: Which species would give the best myoglobin crystals? Initially, Kendrew used horse heart as raw material, but the crystals thus obtained were too small for X-ray analysis. Kendrew realised that a better chance was offered by the oxygen-conserving tissue of diving mammals, because they are rich in myoglobin, which is heavily used as an oxygen store in the muscle, and he got a large chunk of sperm whale meat from Peru. Whale myoglobin gave large crystals with clean X-ray diffraction patterns. However, notwithstanding the great development of X-ray crystallography techniques, for many years it was not possible even in principle to imagine how the structures of crystals so complex could be discovered. Their X-ray patterns contained many thousands of reflections, paralleling the complexity of the molecules themselves. For their interpretation, there was no hope of proceeding by “trial and error” methods, on which X-ray crystallography techniques still largely depended; the first model could never be good enough to provide a useful starting point. So although protein crystallographers discovered many interesting facts about protein crystals, they did not succeed in extracting much information bearing directly on the molecular structure.
Myoglobin gave good X-ray photographs, but for all the above mentioned reasons the images proved impossible to interpret until 1953, when Perutz recalled a strategy that his mentor Bernal had proposed more than a decade earlier: the incorporation of heavy atoms into haemoglobin crystals altered the diffraction patterns produced by X-rays, providing a frame of reference for obtaining the phase relationships of the diffracted waves. Taking X-ray diffraction pictures of crystals identical in shape and molecular structure, but differing in that in one case a heavy atom is present, while in another case it is absent (or replaced by a different heavy atom), then the positions of the diffracted beams do not alter, and only the intensities vary. If the crystals were indeed strictly isomorphous, by comparing the original and modified patterns, it would be possible to determine the location of specific atoms and hence gain important information about the structure of the crystal. The method had been used successfully on smaller molecules. However, nobody thought that it would be possible to apply it to such huge molecules as proteins. Perutz did not give up and found a way to attach mercury atoms to sulphydryl groups on haemoglobin without any change in the oxygen uptake. This indicated that the structure of the molecules most likely had not been altered. He succeeded in crystallising this haemoglobin including heavy mercury atoms, making it possible, in principle at least, to solve the complex X-ray pattern of a protein crystal and to produce a model of the structure of the molecule.
Kendrew applied the isomorphous replacement method to his own research, but he found that myoglobin would not retain mercury atoms, and he was forced to search for other heavy atoms as a substitute, applying a laborious empirical procedure. He was then able to proceed with his collaborators to a study of the three-dimensional structure of the crystal.
At that time, Lawrence Bragg, on his retirement from the Cavendish Professorship, took up the position as Director of the Royal Institution in London, a position previously held by his father. He appointed Kendrew and Perutz visiting readers of the Royal Institution and assembled a team of scientists to work on protein structure. All were now set to work on myoglobin, so that, backed by the group in London, Kendrew now made a relentless attack on the high-resolution structure of this protein. In order to produce a spatial representation of the electron density throughout the crystal, they had to study an incredibly large number of reflections and to calculate the phases. This required, for an unambiguous solution, comparison of several isomorphous heavy-atom replacements in different parts of the molecule. The whole diffraction pattern of a myoglobin crystal consists of at least 25,000 reflections. In 1955, when the three-dimensional work was begun, there were no computers fast enough to calculate Fourier syntheses containing so many terms; besides, the method of heavy-atom replacement was unproved, and it seemed advisable to test it first on a smaller sample of data.
The first application of the isomorphous replacement method was to produce a two-dimensional projection of the structure. But the amount of structural information that could be derived from a projection was almost nil, owing to the high degree of overlap of the elements of so complex a structure. It was immediately clear that to exploit the method they would have to apply it in three dimensions, to produce a spatial representation of the electron density throughout the crystal.
Even if the path was now open for a direct structure determination of haemoglobin and myoglobin, there was still an enormous amount of data to be processed. After the isomorphous replacement method devised by Perutz, the second key tool that allowed the final solving of the structure of haemoglobin and myoglobin was the electronic computer. Crystallographers were among the first scientists to use the first generation of valve electronic computers. In 1951, Cambridge University was one of only three or four places in the world with a high-speed stored-program electronic computer. Kendrew took full advantage of the speed of Cambridge’s EDSAC machine and its more powerful successors, to execute the complex mathematical calculations required to solve the structure of myoglobin. He was actually the first to apply such new tools to the solution of a complex problem in biology.
Nevertheless, even with the EDSAC computer performing the calculations, the research progressed remarkably slowly. Only by the summer of 1957 did Kendrew and his team succeed in creating a three-dimensional map of myoglobin at the so-called “low resolution” of 6 angstroms, which they had planned as the first stage of the project. At this level, it was possible to reveal the general arrangement of the polypeptide chains in the molecule, but not the configuration of the atoms within the chains or that of the side chains surrounding them. They had to measure 400 independent X-ray reflections, from the unlabeled myoglobin crystals and from each of five types of crystal containing heavy atoms. Myoglobin became the first protein to be solved. From the calculated electron density maps, a model of the myoglobin molecule to 6-angstrom resolution was constructed. Calculations completed in the summer of 1957 gave the density of electrons at a large number of points in the crystal, a high electron density being found where many atoms were concentrated. The model contained a number of dense rod-like features that had the dimensions of alpha-helices and made up the bulk of the polypeptide chain. The chain was folded in an irregular manner, certainly much more complicated than had been anticipated. In a pocket in the molecule, there was a dense flattened disc, which presumably was the position of the haeme group with its central iron atom.
The 6-angstrom resolution was too low to show the molecule’s finer features, but by 1960 Kendrew and his team were able to obtain a map of the molecule at 2-angstrom resolution. To achieve a resolution of 2 Angstrom it was necessary to determine the phases of nearly 10,000 reflections, and then to compute a Fourier synthesis with the same number of terms. The Fourier synthesis itself (excluding preparatory computations of considerable bulk and complexity) required about 12 hours of continuous computation on a very fast machine, EDSAC II, at the nearby Mathematics Laboratory, probably the fastest computer in the world then.
The resulting map showed the haeme group in detail, the polypeptide chain and many side chains, including the famous histidine residues that coordinate the haeme. Looking at the map from the appropriate angles, they were able to see this group as a flat object with a region of high density at the iron atom in the centre. There were clear indications that the polypeptide chain indeed assumed a helical shape. Detailed measurement of the spiral density showed that it followed precisely the dimensions of the alpha helix deduced by Pauling and Corey 10 years earlier. In fact, it turned out that about three-quarters of the polypeptide chain in the molecule took the form of straight lengths of alpha helix.
One of the new problems thrown up was how to build a model of a molecule with 1200 atoms and how to compare this with the calculated electron density. Steel rods were set up on a wooden base at distances of about 2 inches (the scale being 2 inches per Angstrom). Electronic density was displayed with coloured clips attached to the rods. An atomic model could be built into this walk-in display by using preformed 'atoms' made of brass rods soldered together with the appropriate valency. These models could be secured by metal joiners representing covalent bonds. The models became known as Kendrew models and (on a smaller scale) were widely used in protein crystallography for the next 25 years. The rather large model of myoglobin that Kendrew built was housed in the disused cyclotron hall in the Cavendish Laboratory (it is now in the Science Museum).
The first glimpse of a protein structure moved Kendrew to liken the moment when he and Perutz first regarded the structure of myoglobin to the discovery of the New World. In that same year 1960, Kendrew was elected a Fellow of The Royal Society. In 1959 he had founded the Journal of Molecular Biology that he ran for many years as Editor-in-Chief. The journal did much to define the emerging discipline of molecular biology.
Because myoglobin has a single chain whereas haemoglobin has four, Kendrew's work with myoglobin progressed more rapidly. The publication of the atomic structure of myoglobin, along with the 6 A structure of haemoglobin by Perutz and his collaborators in Nature in 1960, truly heralded a new era in biology. These papers reported the first solution of the three-dimensional molecular structure of a protein.
They found that both molecules were built from Linus Pauling's alpha helices, but folded and packed together in a complicated manner that never could have been deciphered by any other technique. With structure information in hand they could then explain how haemoglobin in the bloodstream binds and releases oxygen on cue, how it passes its cargo on to the related storage protein myoglobin, and how a single amino acid mutation can produce the catastrophe known as sickle-cell anaemia, described by Pauling and his collaborators in 1949 as the first molecular disease. Perutz and Kendrew also observed that the folding of helices was identical in myoglobin and the two chains of haemoglobin, and this along with the simultaneously evolving new technique of amino acid sequence analysis established for the first time the concept of molecular evolution.
For being the first to successfully identify the structures of complex proteins John Kendrew and Max Perutz were honoured with alacrity by the award of the 1962 Nobel Prize in Chemistry. That same year they moved into the new Medical Research Council Lab of Molecular Biology in Cambridge, with Perutz as director, and Kendrew as deputy director and head of the division of structural studies. Kendrew was later appointed project leader to develop the European Molecular Biology Lab founded in Heidelberg in 1974. He set up the EMBL outstations at DESY (Hamburg) and ILL (Grenoble) for synchrotron light as an X-ray source and for neutron scattering; the DESY outstation was the first in the world to use synchrotron radiation as a source for X-ray diffraction, thus setting the stage for the large-scale use of this powerful technique.
Bibliography
Hagan W. J. Jr (1993) John Kendrew. In Laylin K. J. Ed. In Nobel Laureates in Chemistry 1901-1992 (American Chemical Society and the Chemical Heritage Foundation), pp. 428-433
Holmes K. C. (2001) Sir John Cowdery Kendrew. Biographical Memoirs of Fellows of the Royal Society 47: 312-332
Kendrew, J. C. (1961) The Three-dimensional Structure of a Protein Molecule. Scientific American 205 (6): 96-110
Kendrew J. C. Myoglobin and the structure of proteins Nobel Lecture, December 11.
http://www.nobelprize.org/nobel_prizes/chemistry/laureates/1962/kendrew-lecture.pdf
Strandberg B., Richard E. Dickerson R. E and Rossmann M. G. (2009) 50 Years of Protein Structure Analysis. Journal of Molecular Biology 392: 2-32
Wasson, T. (ed.) (1987) Kendrew, John C. In Nobel Prize Winners, H. W. Wilson Company, New York, pp. 544-546